新闻动态
...domain of the modular xylanase XynA of Thermotoga maritima...
2024-04-28
The Thermostabilizing domain of the modular xylanase XynA of Thermotoga maritima represents a novel type of binding domain with affinity for soluble xylan and mixed‐linkage β‐1,3/β‐1,4‐glucan - Meissner - 2000 - Molecular Microbiology - Wiley Online Library Free Access The thermostabilizing domain of the modular xylanase XynA of Thermotoga maritima represents a novel type of binding domain with affinity for soluble xylan and mixed-linkage β-1,3/β-1,4-glucan Give accessShare full text accessShare full-text accessPlease review our Terms and Conditions of Use and check box below to share full-text version of article.I have read and accept the Wiley Online Library Terms and Conditions of UseShareable LinkUse the link below to share a full-text version of this article with your friends and colleagues. Learn more.Copy URLShare a linkShare onEmailFacebookTwitterLinked InRedditWechat AbstractThermotoga maritima XynA is an extremely thermostable modular enzyme with five domains (A1–A2–B–C1–C2). Its catalytic domain (–B–) is flanked by duplicated non-catalytic domains. The C-terminal repeated domains represent cellulose-binding domains (CBDs). Xylanase domains related to the N-terminal domains of XynA (A1–A2) are called thermostabilizing domains because their deletion normally leads to increased thermosensitivity of the enzymes. It was found that a glutathione-S-transferase (GST) hybrid protein (GST-A1A2) containing both A-domains of XynA can interact with various soluble xylan preparations and with mixed-linkage β-1,3/β-1,4-glucans. GST-A1A2 showed no affinity for insoluble microcrystalline cellulose, whereas, vice versa, GST-C2, which contains the C-terminal CBD of XynA, did not interact with soluble xylan. Another hybrid protein, GST-A2, displayed the same binding properties as GST-A1A2, indicating that A2 alone can also promote xylan binding. The dissociation constants for the binding of xylose, xylobiose, xylotriose, xylotetraose and xylopentaose by GST-A2, as determined at 20°C by fluorescence quench experiments, were 8.1 × 10−3 M, 2.3 × 10−4 M, 2.3 × 10−5 M, 2.5 × 10−6 M and 1.1 × 10−6 M respectively. The A-domains of XynA, which are designated as xylan binding domains (XBD), are, from the structural as well as the functional point of view, prototypes of a novel class of binding domains. More than 50 related protein segments with hitherto unknown function were detected in about 30 other multidomain β-glycanases, among them putative plant (Arabidopsis thaliana) xylanases. It is argued that polysaccharide binding and not thermostabilization is the main function of A-like domains. Introduction Xylans are major hemicellulose components of plant cell walls where they are closely associated with the main structural polysaccharide, cellulose, and with the aromatic polymer, lignin. Xylans have a relatively complex structure based on a non-branched β-1,4-glycosidically linked xylose backbone. Depending on the origin, the backbone structure is substituted to varying degrees with acetyl, l-arabinofuranosyl, glucuronyl and 4-O-methylglucuronyl groups (see 9). The complete decomposition of xylan by microorganisms requires the concerted and synergistic action of a variety of enzymes specific for the different linkages between the xylan building blocks. The two enzymes generally involved in main-chain breakdown are endo-1,4-β-d-xylanase (1,4-β-d-xylan-xylanohydrolase, EC 3.2.1.8) and β-xylosidase (β-d-xyloside-xylohydrolase, EC 3.2.1.37). In addition, accessory enzymes are needed: (i) to cleave glycosidic bonds between l-arabinose or 4-O-methylglucuronic acid side-chains and backbone xylose units (α-l-arabinofuranosidase, EC 3.2.1.55, and α-d-glucuronidase respectively); and (ii) to cleave ester bonds to remove acetyl groups from the backbone of hardwood xylans (acetyl xylan esterase) and to break linkages between xylan and lignin (p-coumaryl esterase, feruloyl esterase). Thermotoga maritima and the closely related species, Thermotoga neapolitana, strictly anaerobic hyperthermophiles capable of growth at temperatures up to 90°C, are the most thermophilic xylan-degrading bacteria currently known. They appear to possess similar xylan degradation systems. T. maritima is able to produce a high-molecular mass xylanase (120 kDa, XynA), a low-molecular mass xylanase (40 kDa, XynB), a β-xylosidase, an α-l-arabinofuranosidase and an α-d-glucuronidase (48; 49; 37; unpublished data). In addition, the T. maritima genome sequencing project (32) has revealed the presence of a putative acetyl xylan esterase gene. Thus, this organism appears to possess all the important enzyme components for the efficient breakdown of complex xylans, which is in accordance with its ability to rapidly grow on these polysaccharides. The main goal of this study was to elucidate the function of the N-terminal domains A1 and A2 of the modular T.maritima xylanase XynA. These domains belong to a domain type whose role to date has remained largely obscure. A variety of cellulolytic and hemicellulolytic enzymes have multidomain structures with one (in some cases two) catalytic domain(s) and one or more non-catalytic domains of often unknown function (see 17; 43). Comparative amino acid sequence analyses have revealed that members of the same family of catalytic domains can be connected to different types of non-catalytic domains and vice versa; members of one family of non-catalytic domains can be connected to different types of catalytic domains. It seems clear that substantial shuffling of domain-encoding sequences by genetic mechanisms that are not known in detail has led to the large variety of multidomain glycosyl hydrolases (see 18) known today. The 1059 amino acid, about 120 kDa xylanase XynA of T. maritima, also has a modular structure of this kind, with five domains in the order A1–A2–B–C1–C2. Its N-terminal signal peptide is followed by two related ∼150 residue domains (A1–A2), a ∼ 340-amino-acid catalytic domain belonging to glycosyl hydrolase family 10, a short proline-rich linker sequence and two related ∼ 170 residue domains (C1–C2). Domain C2 and, based on its amino acid sequence similarity to C2, possibly also C1 represent cellulose-binding domains that confer upon XynA the ability to reversibly bind to microcrystalline cellulose (49). The N-terminal domains of XynA have been found to be important for enzyme stability. At 75°C, XynA in 50 mM Bis-Tris pH 6.2–0.25 M NaCl resists thermoinactivation for more than 2.5 h. A XynA deletion derivative lacking both N-terminal domains (XynAΔN) and a derivative devoid of all the non-catalytic domains (XynAΔNC) were significantly less thermostable than the full-length enzyme, whereas the thermostability of the derivative XynAΔC was similar to full-length XynA. These results suggested that the N-terminal part of the enzyme containing domains A1 and A2 is more important for thermostability than the C-terminal part (49). Similarly, deletion of A-like domains from other xylanases has resulted in enzyme derivatives that were much more susceptible to thermoinactivation than the full-length enzymes (26; 15). As a consequence of these observations, these domains often are called thermostabilizing domains (26; 15; 8; 51; 25; 31). We now show that the A-domains of T. maritima XynA interact with soluble plant cell wall polysaccharides and derivatives thereof, indicating that their main function is substrate binding. These domains and most likely also related domains of other xylanases represent members of a novel type of soluble β-glycan-binding domain. Separation of the domains of modular glycosyl hydrolases from each other has demonstrated that they can often function independent of each other (see 17; 43; 49; 47). In order to elucidate the function of the N-terminal domains A1 and A2 of the T. maritima xylanase XynA, we constructed hybrid proteins containing A1 and A2 of XynA fused to the C-terminus of Schistosoma japonicum glutathione S-transferase (GST). With this GST protein fusion approach we wanted to avoid the possible interference of the C-terminal cellulose-binding domains and also of the catalytic domain of XynA with the subsequent polysaccharide–protein binding assays. Specific primers matching the approximate borders of the region coding for A1–A2 were selected on the basis of the nucleotide sequence of the xynA gene (EMBL accession no. Z46264) as described in the Experimental procedures. The gene segment was amplified via the PCR and joined in frame to the 3′ end of the S. japonicum glutathione S-transferase gene of vector pGEX-4T-2, resulting in the plasmid pGST-A1A2. The same strategy was used to construct expression plasmids with in frame fusions of the 3′ end of the GST open reading frame (ORF) and the xynA regions encoding either A1 or A2, resulting in the plasmids pGST-A1and pGST-A2 respectively (see Experimental procedures). The latter fusions were made in order to see if the A-domains could also function independent of each other. Plasmids pGST-A1A2, pGST-A1 and pGST-A2 encode (polypeptide designation and amino acid residues of full-length XynA in brackets) GST fused to the domains A1–A2 (GST-A1A2; residues 31–362 of XynA), A1 (GST-A1; residues 31–199 of XynA) and A2 (GST-A2; residues 198–354 of XynA). The hybrid genes were expressed via IPTG-induction after transformation into Escherichia coliXL1-Blue. Crude cellular extracts were prepared and analysed by SDS–PAGE. Highly expressed protein bands were detected with sizes of about 63 kDa, 44 kDa and 46 kDa (not shown), which corresponds well to the sizes expected for the fusion proteins GST-A1A2, GST-A1 and GST-A2 respectively. All three proteins displayed glutathione S-transferase activity, indicating that fusion of the enzyme with the A-domains of XynA did not result in misfolding at least of the GST part of the hybrid proteins. The GST fusion proteins, which under optimized induction conditions were expressed at levels of between about 25% and 45% of soluble cellular proteins, were purified by affinity chromatography on glutathione Sepharose 4B. The yields obtained were 67% for GST-A1A2 and nearly 100% for GST-A1 and GST-A2. The level of protein purity reached was estimated to be higher than 95% (data not shown). Detection of polysaccharide binding by native affinity polyacrylamide gel electrophoresis (NAPAGE) and determination of the binding substrate specificity NAPAGE is based on the retarded mobility of a protein containing polysaccharide-binding domains during native polyacrylamide gel electrophoresis if a soluble binding substrate is included in the separation gel. Purified GST-A1A2 was separated on non-SDS polyacrylamide gels containing xylan or a variety of other soluble polysaccharides at a final concentration of 0.1%, as described in the Experimental procedures. When compared with a reference gel without polysaccharide, significant retardation of the hybrid protein was found to occur in the presence of oat spelts xylan, birchwood xylan, 4-O-methylglucuronoxylan, DMSO xylan, Rhodymenia xylan, wheat straw xylan, barley β-glucan, lichenan, methyl cellulose and hydroxyethyl cellulose (Fig. 1). Various other β-glycans, as well as all tested α-glycans had no effect on the mobility of GST-A1A2 (summarized in Table 1). Appropriate controls in each gel demonstrated that glutathione S-transferase without a C-terminal extension did not interact with the polysaccharides included into the gels (see Fig. 1, lane 2 of each gel). GST-A2 behaved like GST-A1A2 in the NAPAGE assays while the mobility of GST-A1 was not affected by the various polysaccharides tested (Fig. 1 and Table 1). It should be kept in mind that the polysaccharide substrates used in the NAPAGE gels differ in molecular weight and structural complexity (e.g. the type of substituents on the xylan backbone and the substitution degree is variable, depending on the origin of the xylan; see 9). Therefore, the obvious differences in mobility shift observed should not be over interpreted, i.e. when comparing the results obtained at a given ligand concentration with two polysaccharide ligands that both interact with A1A2 or A2, one can not judge with confidence that an increased retardation during electrophoresis definitely indicates a higher binding affinity. As a consequence, the mobility shift experiments summarized in Fig. 1 and Table 1 should be taken as purely qualitative results merely proving either interaction or no interaction. Native affinity polyacrylamide gel electrophoresis (NAPAGE) analysis of GST-A1A2, GST-A1 and GST-A2. Purified protein samples were separated in non-SDS 7.5% polyacrylamide gels containing 0.1% (w/v) of DMSO-xylan (B), Rhodymenia xylan (C), barley β-glucan (D) or hydroxyethyl cellulose (E). A gel without added polysaccharide served as a reference (A). For experimental details see the Experimental procedures. The order of sample application was the same in all gels: lane 1, standards for native polyacrylamide gel electrophoresis (MW-ND-500, Sigma, Deisenhofen, Germany); lane 2, GST; lane 3, GST-A1A2; lane 4, GST-A1; lane 5, GST-A2. Table 1. Interaction of hybrid proteins containing the N-terminal domains of XynA with soluble polysaccharides. β-1,3-d-galactose backbone with β-1,6-and β-1,4-sidechains of arabinose and galactose β-1,4-linked d-glucose polysaccharide hydroxyethyl substituents, ‘medium viscosity’ β-1,3-linked d-glucose polysaccharide with some β-1,6-bonds and 50% terminal mannitol . Suppliers: BDH Chemicals, Poole, UK; Fluka, Buchs, Switzerland; Sigma, Deisenhofen, Germany; Wako Pure Chemical Industies, Kyoto, Japan. . Interaction as judged from altered mobility in NA–PAGE analysis: +, significant band shift if polysaccharide was included in the separation gel; –, mobility in gel not retarded. We tested if the C-terminal domain C2 of XynA, which had previously been identified as a cellulose-binding domain (49), would interact with soluble xylan. For this purpose, a hybrid protein consisting of GST and domain C2 was recombinantly produced and purified by affinity chromatography on a cellulose column as described before (49), and was subjected to NAPAGE analysis in 0.1% xylan-containing native polyacrylamide gels in the same way as described above. No interaction between xylan and GST-C2 could be detected (data not shown). NAPAGE in the presence of increasing concentrations of polysaccharides in the separating gels was carried out in order to obtain apparent dissociation constants for the binding of GST-A2 to barley β-glucan and oat spelts xylan (Fig. 2). Using this method, the dissociation constant for β-glucan was determined to be about 55 µg ml−1. Inclusion of low concentrations (between 1 and 10 µg ml−1) of oat spelts xylan in the gel demonstrated that a significant interaction between the protein and the polysaccharide took place even at the lowest concentration tested, i.e. 1 µg ml−1 xylan. However, a strong smear of the protein band was observed. Presumably, the complex nature of xylan poses a problem here: xylan is not as uniform in structure as other polysaccharides for which quantitative binding data have often been determined (e.g. cellulose or dextran). The fact that there are different substituents along the xylan backbone could result in binding of the xylan-binding domain to different regions with different affinities. Also, the presence of charged groups (4-O-methylglucuronic acid), which could result in electrophoretic mobility of (part of) the polysaccharide, as well as heterogeneity in chain length of oat spelts xylan could complicate the interpretation of the NAPAGE data. Nevertheless, the data obtained indicate that the dissociation constant for this ligand is considerably lower than for β-glucan. NAPAGE analysis of GST-A2 at various concentrations of barley β-glucan or oat spelts xylan. In each of a series of 7.5% polysaccharide-containing polyacrylamide gels, a 3 µg sample of GST-A2 was applied next to a lane containing marker proteins. For the β-glucan gels, the reciprocal relative migration distance of GST-A2 (1/r) was plotted against the polysaccharide concentration. A negative value of the dissociation constant is obtained as the intercept of the regression line on the abscissa. In order to obtain an insoluble binding substrate that could be used for affinity chromatography of proteins with A-like domains, and for a more detailed study of the binding properties, xylan was immobilized by covalent coupling to the commercially available vinyl-activated gel matrix Mini-Leak High (Kem-En-Tec) as described in Experimental procedures. Binding of the hybrid protein GST-A1A2 to the immobilized xylan was demonstrated by testing the retention of the hybrid protein on a column packed with Mini-Leak High − xylan. The result of a typical binding experiment is shown in Fig. 3. GST-A1A2, which had bound to the column matrix, could be eluted with a 0.5% oat spelts xylan solution (see Fig. 3). GST alone had no affinity for the xylan column (not shown). The result that immobilized xylan can be used as an affinity matrix for purification via binding and subsequent elution (Fig. 3) indicates that binding of the xylan binding domain (XBD) to xylan is reversible. Xylan affinity chromatography of GST-A1A2 on a Mini-Leak High − xylan column. A crude cell-free lysate of the E. coli strain XL1-Blue(pGST-A1A2) expressing the fusion protein GST-A1A2 was loaded onto the column in 10 mM Bis-Tris pH 6.2, 1 M NaCl. After 2 h at room temperature, proteinsthat did not adhere were washed from the column matrix and bound proteins were eluted with 0.5% oat spelts xylan as described in Experimental procedures. Lane 2 of the 10% SDS–PAGE gel contains 15 µg crude extract before application to the column, while the same amount of the corresponding material after having passed through the column without binding was applied to lane 3. Next, 2 µg of proteins eluted with 0.5% xylan were separated in lane 4. Note that as a resul of high-level expression a protein band with a molecular mass corresponding well with the expected size of the fusion protein (63 kDa) was clearly visible in the crude extract (lane 2). This band disappeared after passage of the sample through the column (lane 3) and could be released from the column with 0.5% xylan solution (lane 4). Glutathione S-transferase not fused with an A-domain did not adsorb to immobilized xylan (not shown). The immobilized xylan was used as a binding substrate for further characterization of the tandem xylan-binding domains A1A2 of XynA. The purified hybrid protein GST-A1A2 was used for these experiments. In general, defined amounts of GST-A1A2 and immobilized xylan (Mini-Leak High − xylan) were mixed in buffer and incubated, allowing sufficient time for binding. Then the amount of non-adsorbed protein in the supernatant was determined either via its GST activity or by measuring the protein concentration. Binding was not dramatically affected over a broad range of pH values (Fig. 4), which is a feature also characteristic of many cellulose-binding domains (CBDs) (33; see 44). The temperature also did not have a dramatic effect on xylan binding (Fig. 4). Acidic pH values (pH 5.5 or less) and high temperatures of 80°C or higher led to precipitation of the hybrid protein, probably mainly due to the GST portion. The presence of salt was crucial for efficient binding of the A-domains to immobilized xylan (Fig. 4). Near-maximum binding was observed at NaCl concentrations above 200 mM. Very high concentrations of NaCl (up to at least 3 M) had no adverse effect on binding. Effect of pH (A), temperature (B) and NaCl concentration (C) on the binding of GST-A1A2 to immobilized oat spelts xylan. Binding experiments were carried out as described in Experimental procedures by adding a defined amount of fusion protein to a suspension of immobilized xylan. After incubation, binding was quantified by measuring the residual protein amount (pH and temperature dependence) or the residual GST activity (NaCl dependence) in the supernatant. For each pH, temperature or NaCl concentration tested a reference without binding substrate was measured. The protein content or GST activity, respectively, of the reference samples were defined as 100%. The influence of various mono-, di- and soluble polysaccharides on the binding of GST-A1A2 to immobilized xylan was investigated by measuring the effect of the presence of these saccharides on binding efficiency in the standard binding assay (see Experimental procedures). Various monosaccharides (d-xylose, d-glucose, d-galactose, l-arabinose, d-mannose, d-glucuronic acid, at a final concentration of 200 mM), disaccharides (maltose, lactose, cellobiose, at 100 mM), as well as the polysaccharides arabinogalactan, mannan and a cellodextrin mixture (at 0.4% each) had no effect on binding (data not shown). All polysaccharide substrates tested that resulted in significant retardation of mobility in the NAPAGE test system (see above, and Fig. 1 and Table 1) also antagonized binding to immobilized xylan, i.e. methyl cellulose, oat spelts xylan and Rhodymenia xylan (tested at final concentrations between 0.2% and 1%) (Table 2). Oat spelts xylan preparations that had been enzymatically digested with xylanase were less effective than untreated oat spelts xylan in preventing the binding of GST-A1A2 to immobilized xylan. This result suggests that polymeric xylan is a better binding substrate than small xylan-derived oligosaccharides. At a concentration of 1% soluble oat spelts xylan, nearly complete (85%) inhibition of binding to the immobilized xylan was reached. Table 2. Effect of saccharides on binding of GST-A1A2 to immobilized xylan (Mini-Leak High − oat spelts xylan). Saccharides were added to standard xylan-binding assays at the final concentrations listed in the table. After incubation, the GST activity in the supernatant was compared with references without immobilized xylan (0% binding, equivalent to 100% inhibition of binding) and without saccharide (defined as 100% binding, equivalent to 0% inhibition of binding). . Only the soluble components; a 5 × solution of the polysaccharide was prepared by autoclaving for 10 min and 200 µl of a 10 000 × g (15 min, 20°C) supernatant was added to the binding assay (1 ml total volume). Where indicated, samples were treated with 40 U XynA ml −1 at 75°C before centrifugation. According to thin layer chromatography and HPLC analysis (data not shown), xylan samples digested with xylanase for 18 h contained mainly xylobiose, xylotriose, at least five different substituted xylooligosaccharides and small amounts of xylose and xylotetraose, while samples digested for merely 5 h contained significantly more xylotetraose and xylopentaose and several additional longer substituted xylo-oligosaccharides. The standard immobilized-xylan binding assay was used in order to determine whether the A-domains were able to interact with the insoluble cellulose substrates Avicel PH105 (Serva) and ball milled Avicel. Neither GST-A1A2 nor GST-A2 had significant binding affinity for these microcrystalline cellulose preparations (data not shown). This result is in agreement with previous results, which had shown that a XynA derivative with a C-terminal deletion (lacking the CBD domains but still containing both A-domains and the catalytic domain) was unable to bind to Avicel (49). Saccharide binding was found to influence the fluorescence emission spectrum of the GST-A2 hybrid protein (Fig. 5). In the native protein, aromatic residues are partially exposed to the solvent, as indicated by the maximum of fluorescence emission at 344 nm (40). Upon sugar binding, a shift to 338 nm and a decrease of intensity of ≈ 10% occurs as a result of changes in the environment of the aromatic residues (either involvement of aromates in sugar binding or conformational change). The spectrum of GST lacking the A2 fusion part was not changed by the addition of xylo-oligosaccharides (data not shown). Binding of xylo-oligosaccharides to GST-A2 at 20°C. The protein concentration was 0.22 µM in 50 mM sodium phosphate, 100 mM NaCl, pH 6.4. A. Fluorescence quench at λem = 366 nm (λexc= 280 nm) of 0.22 µm GST-A2 with with xylopentaose (●). Insert, fluorescence emission spectra (λexc= 280 nm) of unliganded GST-A2 (—) and the xylopentaose-GST-A2 complex (- − -). B. Relative change of fluorescence at λem = 366 nm (λexc= 280 nm) of 0.22 µM GST-A2 with xylotriose (▾), xylotetraose (○), as well as with xylopentaose (●). Lines represent fits assuming one binding site per monomer. Fluorescence titration of GST-A2 with various oligosaccharide ligands was carried out in order to further characterize the substrate binding properties of the A-domains of XynA (Fig. 5). Dissociation constants for the binding of xylo-oligosaccharides to GST-A2 were determined with xylose through xylopentaose at 20°C as described in Experimental procedures. The dissociation constants (Kd values summarized in Table 3) decreased from millimolar to micromolar concentrations with increasing chain length of the binding substrate. The preference for long-chain over short-chain ligands is in line with the observation that extensively xylanase-digested xylan was less efficient than undigested xylan in inhibiting the binding of GST-A1A2 to immobilized xylan (see above, and Table 2). Table 3. Dissociation constants of binding of xylo-oligosaccharides to GST-A1A2 determined at 20°C. Amino acid sequence similarity between the A-domains of XynA and other glycosyl hydrolases The available sequence databases were scoured for proteins with similarity to the A-domains of XynA. More than 50 representatives of A-like domains were detected in numerous (about 30) other enzymes, mostly xylanolytic enzymes, of various organisms (Fig. 6). On the basis of these results a new XBD family is proposed. The degree of similarity between the domains is significant, but often low. Apart from the thermostabilizing role (see Discussion below), which has been observed for a few of the A-like domains, the function of the domains listed in Fig. 6 was not known to date. It seems likely that in these cases the A-like domains also represent XBDs. Low-level similarity was also detected between the A-domains and two domains C-terminal of the catalytic domain of the Thermoanaerobacterium polysaccharolyticum mannanase CelA, which recently was shown to have affinity to a cellulose-based column matrix (7), indicating that these domains may also belong to the new XBD family. An interesting feature of the alignment of A-like domains is the presence of five conserved aromatic residues (Fig. 6). In other carbohydrate-binding proteins, hydrogen bonding to sugar hydroxyls as well as van der Waals interactions (especially stacking type van der Waals forces between sugar units and aromatic amino acid residues) contribute to the sugar-binding protein association (see 46; 36; 24) and aromatic residues have been shown to be involved in the binding of CBDs to cellulose (35; 13; 6; 45). Amino acid sequence similarity of A-like domains. The multiple sequence alignment was calculated with the program clustalw. Positions with a high degree of prevalence of particular amino acids or conservative exchanges are shaded to guide the eye and are marked in the consensus line at the bottom as letters or ^ symbols respectively. Identity and similarity percentages with the domains A1 and A2 of T. maritima XynA in the table next to the alignment were calculated from pairwise alignments with the program gap and are listed in the table. Numbers in brackets indicate low-quality or uncertain alignments. Possible subfamilies of A-like domains were defined by calculating a neighbour joining tree on the basis of the multiple sequence alignment (vertical brackets). The sequence designations used here are composed of an abbreviation for the organism, followed by the enzyme/gene designation, followed by a number in cases where more than one A-like domain is present in the enzyme. The enzyme designation ‘put’ stands for putative protein with similarity to xylanases. Abbreviations for organisms: Atha, Arabidopsis thaliana;Ttsp, Thermotoga species; Tnea, Thermotoga neapolitana;Tmar, Thermotoga maritima;Casp, Caldocellulosiruptor species; Csac, Caldocellulosiruptor saccharolyticus;Athe, Anaerocellum thermophilum;Tthe, Thermoanaerobacterium thermosulphurigenes;Tasp, Thermoanaerobacterium species; Tsac, Thermoanaerobacterium saccharolyticum;Cthe, Clostridium thermocellum;Rfla, Ruminococcus flavefaciens;Rusp, Ruminococcus species; Ralb, Ruminococcus albus;Basp, Bacillus species; Acav, Aeromonas caviae;Tpol, Thermoanaerobacterium polysaccharolyticum;Cfim, Cellulomonas fimi;Cste, Clostridium stercorarium. Many cellulases and xylanases contain CBDs, which promote a close interaction between the enzymes and cellulose and can assist in substrate hydrolysis in different ways (12; 10; 16; 38; 2; 29; 4; 5; 47). Certain CBDs can interact with soluble cello-oligosaccharides in addition to their regular insoluble substrates (49; 44; 42). We have now identified a novel binding domain type with affinity for soluble β-glycans but not for microcrystalline cellulose. The experimental technique used in this study, NAPAGE, which is reminiscent of band shift assays commonly used in the analysis of protein–DNA interactions, represents an elegant method for analysis of the interaction between proteins and soluble polysaccharides. In our case, various xylans, barley β-glucan, lichenan, methyl and hydroxyethyl cellulose, which were included in native polyacrylamide gels, significantly retarded the mobility of the purified hybrid protein GST-A1A2 during electrophoresis (see Table 1). Further proof for A1A2 as a xylan-binding polypeptide was obtained by showing that GST-A1A2 bound to immobilized xylan. Also, NAPAGE experiments with GST-A2 showed that domain A2 by itself promoted efficient xylan binding and was not dependent on the presence of A1. Its interaction with various xylans, its inability to bind microcrystalline cellulose, its high affinity for medium- and long-chain xylo-oligosaccharides (in the micromolar range for DP ≥ 4) and the detection of homologous domains in many xylanases (see Fig. 6, and Discussion below) justifies the designation of A2 as a XBD. Although GST-A1 did not interact with xylan under our assay conditions, A1 (about 41% similarity/30% identity with A2) could also represent a XBD. Possible reasons for the failure of GST-A1 to bind xylan could be: (i) the domain may be misfolded in the fusion protein; (ii) A1 may not be able to bind independently of A2; and (iii) A1 may have a different binding specificity than A2. As a consequence of identifying the function of the A-domains of T. maritima XynA, we can now conclude that XynA is an enzyme with two different kinds of binding domains specific for two different kinds of plant cell wall polysaccharides: one type of binding domain (C-terminal) is specific for an insoluble polysaccharide (crystalline cellulose) which is not a substrate for the catalytic domain of XynA, whereas the other type (N-terminal) is specific for soluble polysaccharides (xylan, mixed-linkage β-glucan), which at the same time are also substrates for the catalytic domain. When thinking about the possible roles of A-like XBD domains, their binding specificity is of particular interest. In contrast to the C-terminal CBDs of XynA (49), the N-terminal XBDs had no affinity for insouble microcrystalline cellulose, but various xylan preparations were identified as binding substrates for A2 and A1A2. In addition to xylans, certain mixed-linkage β-1,3/β-1,4-glucans (barley β-glucan, lichenan) were recognized. The same holds true for the catalytic specificity of the catalytic domain of XynA, which hydrolyses barley β-glucan and lichenan in addition to its preferred substrate xylan (48). At present it is unknown if the catalytic activity of XynA and also the binding specificity of the A-domains towards mixed-linkage β-glucans is of physiological importance. NAPAGE analysis revealed a Kd value for the binding of GST-A2 to β-glucan of about 55 µg ml−1. Although it was not possible to determine the Kd for oat spelts xylan with this method, the NA–PAGE data shown in Fig. 2 indicate a much higher affinity of the fusion protein for xylan than for β-glucan, which provides further support for the designation of A2 as a xylan-rather than a β-glucan-binding domain. The fact that a non-substituted xylan (from Rhodymenia) was a binding substrate shows that the xylan backbone and not the side-chains (acetyl, methyl-glucuronyl, arabinosyl groups) are recognized by the binding domain. A more detailed picture of the xylan-binding region of A2 can be derived from quantitative ligand binding data for the series of non-substituted xylo-oligosaccharides. The Kd values measured with fluorescence quench decreased by almost four orders of magnitude from millimolar to micromolar concentrations in the order xylose ≫ xylobiose ≫ xylotriose ≫ xylotetraose xylopentaose, meaning that binding affinity increased with increasing chain length of the ligand. If one binding site per GST-A2 monomer is assumed, these results point to a binding site that can bind xylo-oligosaccharides with a length of (at least) five xylose residues. This is similar to the situation of the N-terminal cellulose-binding domain of Cellulomonas fimi CenC whose cellulose-binding site has been proposed to span approximately five sugar (glucose) residues (24). The concept that enzymes that act on insoluble polymeric substrates such as crystalline cellulose, insoluble β-1,3-glucan, chitin, granular starch or poly β-hydroxybutyrate (PHB) often possess substrate binding domains is well established (see 23; 47). On the other hand, it is generally believed that substrate-binding domains are not required for the degradation of soluble substrates. Our results indicate that at least in the case of plant cell wall depolymerases this assumption should not be taken for granted, because the A-domains of XynA clearly interact with soluble β-glycans. One must keep in mind, however, the complex nature of the plant cell wall, where different polymers, including cellulose, hemicelluloses and lignin are closely associated with each other. Thus, much of the xylan encountered by plant cell wall depolymerases may in fact be insoluble as a result of covalent and/or non-covalent attachment to other wall components. Perhaps domains like the N-terminal domains of XynA aid in the breakdown of xylan present in such natural mixed-polymer substrates. In this case, one of the major roles anticipated for insoluble polysaccharide-binding domains, i.e. to concentrate the enzyme near the surface of the substrate (see 41; 43; 4; 28) may also hold true for XBDs. The results reported here have implications for several other glycosyl hydrolases. A survey of the available sequence databases revealed the presence of A-like domains in numerous (about 30) other enzymes from various organisms (Fig. 6). Most of the enzymes with this domain type are xylanolytic enzymes. Thus, the A-domains of XynA can be regarded as the prototypes of a new large family of binding domains, and it seems likely that many if not all of the A-like domains of other enzymes will prove to have affinity for xylan and thus are also XBDs. Until now, XBDs have been regarded as rare exceptions among the non-catalytic domains of glycanases. To our knowledge there are merely two reports of non-catalytic domains with specific xylan-binding capacity. In both cases, insoluble xylan preparations were used as the binding substrate (3; 14). Figure 7 schematically summarizes the structures of some enzymes containing A-like domains. Interestingly, most of the enzymes with A-like domains are multidomain xylanases, which underscores the idea that these domains may play a functional role in xylan degradation. The majority of enzymes with A-like XBD domains have catalytic domains belonging to glycosyl hydrolase family 10, but combinations with other catalytic domains are also found. The XBDs are often present as two repeats in tandem, but single copies are possible (e.g. in Ruminococcus xylanases) as well as up to four copies (Arabidopsis thaliana polypeptide similar to endoxylanases). In most cases, the XBD domains are located on the N-terminal side of the catalytic domain while CBD domains, if present, are located on the C-terminal side. It is intriguing that a large number of xylanases appear to have binding domains for two different polysaccharides, i.e. for insoluble cellulose and for xylan/β-glucan. The reason for this domain composition surely must lie in the complex structure of the plant cell wall, but the following question remains to be answered: do specific combinations of different binding domains direct an enzyme to a specific complex cell wall microenvironment or do they simply extend the range of possible binding sites, thus making the enzyme more versatile? Modular structure of a selection of enzymes containing A-like domains related to the N-terminal XBD domains of T. maritima XynA. Boxes representing domains with significant amino acid sequence similarity are filled in with the same pattern. Abbreviations: CBD, cellulose binding domain or CBD-homologous domain; GHF, glycosyl hydrolase family; S-like, S-layer-like (SLH) repeats, XBD, xylan-binding domain or XBD-homologous domain; DO, dockerin repeat for cellulosome association. White boxes are protein segments with unknown function. For clarity, the location of linker sequences present in some of the enzymes were omitted. Strikingly, A-like domains are also present in A. thaliana proteins with amino acid sequence similarity to xylanases (see Figs 6 and 7), the sequences of which have become available in the course of the Arabidopsis genome sequencing project. This suggests an important role for this domain type not only in plant cell wall degradation by bacteria but also in cell wall metabolism in plants. In several cases, xylanases with A-like domains were reported to be less stable upon deletion of these domains (see Introduction), leading to their designation as thermostabilizing domains (26; 15; 8; 25; 31). In the light of the discovery that the A-domains of T. maritima XynA are polysaccharide-binding domains, the role of A-like domains as specific thermostabilizing domains is questionable. The following points argue against a general stabilizing role for these domains: (i) although the observed destabilization of xylanases after removal of the A-domains is indisputable, it is not really surprising that deletion of about one third of a polypeptide can severely affect its stability. (ii) A-like domains are present in enzymes with strikingly different domain composition and domain organisation (see Fig. 7). It is difficult to comprehend why and how a single domain type should fulfil a stabilizing role in such a broad range of polypeptide contexts. Also, why is this domain type apparently restricted to plant cell wall-degradative enzymes? (ii) A-like domains are found not only in extreme thermophiles, but also in enzymes from mesophilic bacteria, i.e. Ruminococcus, Aeromonas and Cellulomonas, and even in plant enzymes. (iv) Finally, when considering our present knowledge about the mechanisms of protein stabilization (see 22; 21) it seems to be economically unreasonable to carry on the burden of synthesis and genetic maintenance of a large protein domain (~150 residues for one A-domain) for the sole purpose of stabilization. In conclusion, it appears unlikely that the A-domains of XynA or related enzymes evolved as thermostabilizing domains. More probably, these domains evolved as XBDs that fortuitously sometimes also are important for thermostability of the whole enzymes. Strain MSB8 (DSM 3109) is the type strain of T. maritima (20). E. coli strains used for DNA manipulations were JM83 (50) and XL1-Blue (Stratagene). E. coli strains were routinely grown in Luria broth (LB, 10 g of peptone, 5 g of yeast extract, 5 g of NaCl ad 1000 ml of deionized water, pH 7.2) supplemented with 100 µg ml−1 ampicillin where appropriate. T. maritima strain MSB8 was grown anaerobically in Difco Marine Broth medium no. 2216 (Difco) supplemented with 0.25% soluble starch or xylose (Merck), 0.5% Na2S, 0.0001% resazurin, pH 7.0. For large batch cultures, cells from a fresh overnight culture were inoculated into a reduced medium consisting of 0.5% peptone, 0.1% yeast extract, 0.25% soluble starch or xylose, 1% (v/v) Marine Broth, 3% NaCl, 0.5% Na2S, 0.0001% resazurin dissolved in tap water and preheated to 80°C. Plasmid DNA isolation, transformation of E. coli, and DNA modifications were carried out with standard techniques (1; 39). Nucleotide sequence determination was carried out via the dideoxy chain termination method, using specific sequencing primers flanking the DNA regions of interest. For sequencing and detection, the DIG Taq DNA sequencing kit (Boehringer Mannheim), custom-made digoxigenin-labelled oligonucleotide primers (MWG) and the DIG detection kit (Boehringer Mannheim) were used in combination with a Hoefer TwoStep direct blotting apparatus (Serva). Amplification of DNA fragments encoding the N-terminal domains of XynA via the PCR was performed using Pfu polymerase (Stratagene) or a Taq/Pwo polymerase mixture (Expand high fidelity PCR system, Roche Molecular Biochemicals) with the reaction buffers recommended by the suppliers. Reaction mixtures (100 µl) contained the four dNTPs (200 µM), 50 pmol of each primer, 10–100 ng of pUCXyl1 (a pUC-based high-copy vector containing the complete T. maritima xynA gene; 49) as the template DNA, 10 µl of 10 × reaction buffer and enzyme. Initial denaturation of the DNA was carried out at 94°C for 3 min before starting the reaction by the addition of polymerase enzyme. The PCR cycles were: 30 cycles of 94°C for 30 s, 63°C for 2 min and 72°C for 4 min for amplification of A1A2; 30 cycles of 94°C for 30 s, 60°C for 30 s and 72°C for 45 s for amplification of A1 or A2. After the first 10 cycles, the elongation period was increased by 10 s after each cycle. The amino acid sequences used for alignments were retrieved from the SWISS-PROT and PIR data bases or as translates of DNA sequences from the EMBL nucleotide sequence database. Similarities between protein sequences were calculated with the UWGCG program gap (11). The significance of low-level similarity between two amino acid sequences was evaluated by comparing the quality value of the pairwise alignment calculated by gap with the average quality based on 10 randomizations. Multiple alignments of amino acid sequences were accomplished with the aid of the program clustalw (19) and edited manually for maximum fit. The vector pGEX-4T-2 (Amersham-Pharmacia) was used for the construction and expression of the hybrid proteins used in this study. pGEX-4T-2 carries the lacIq repressor gene for control of the Ptac promoter upstream of the coding region for Shistosoma japonicum GST. At the 3′ end of the GST-encoding sequence there are a series of unique restriction sites for the construction of GST-gene fusions. The following primers were used for the PCR-aided amplification of A1A2, A1 and A2 during the construction of plasmids encoding glutathione S-transferase-XynA protein fusions: A1A2-v, 5′-GCCGGAATTCCAGCATCGGGTGTTCTGAGTTTCG-3′; A1A2-r, 3′-CGTCTCTATTTCTAACTTTACCTTGCTACTGGGCCCACTTG-5′; A1-v, 5′-GCCGGAATTCCAGCATCGGGTGTTCTGAGTTTCG-3′; A1-r, 3′-CGAGGTTTTCTGAGCTCGGGTT-TCCGA-5′; A2-v, 5′-GGTTCAGGGAATTCCTCCAAAAGAATCGG-3′; and A2-r, 3′-GTTGAAGGCAGCTGTATTTCTAACTTTACC-5′. The PCR products obtained were cut with EcoRI+SmaI (for A1A2), EcoRI+XhoI (for A1) or EcoRI+SalI (for A2), and inserted into pGEX-4T-2 treated with the same combinations of restrictions endonucleases, thus yielding the plasmids pGST-A1A2, pGST-A1 and pGST-A2. These plasmids contain the S. japonicum GST gene fused in frame with the xynA gene regions encoding domains A1A2, A1 and A2 respectively. The correctness of the gene fusions was checked by nucleotide sequence analysis. In pGST-A1A2, pGST-A1 and pGST-A2, the hybrid genes are under the transcriptional control of the Ptac promoter that itself is regulated via the product of the lacIq gene. Owing to the mode of construction, the expressed fusion proteins GST-A1A2, GST-A1 and GST-A2 contained additional non-XynA amino acid residues (11, 9 and 10 residues respectively) at their C-terminal ends. The plasmids pGST-A1A2, pGST-A1 and pGST-A2 were transformed into E. coli strain XL1-Blue for subsequent high-level expression. Determination of protein concentrations and SDS–PAGE were carried out as described before (27). The Micro BCA Protein Assay Reagent Kit (Pierce) or the microassay of the Protein Assay Kit (Bio-Rad) were used to measure low protein concentrations in the course of xylan-binding assays. For determination of GST activity, 100 µl of 1 M potassium phosphate buffer pH 6.5 in a cuvette was mixed with an enzyme sample and double distilled water was added to 980 µl. The reaction was started by the addition of 10 µl each of 100 mM reduced glutathione and 100 mM 1-chloro-1,4-dinitrobenzene and colour development was followed at 340 nm for 5 min. One unit of GST activity results in an increase of absorbance of 1.0 in 1 min at 20°C. For high-level expression of the fusion proteins in the recombinant E. coli strains XL1-Blue(pGST-A1A2), XL1-Blue (pGST-A1) and XL1-Blue(pGST-A2), the strains were grown in dYT [XL1-Blue(pGST-A1A2)] medium or LB medium (the other strains) supplemented with 100 µg ml−1 ampicillin at 37°C with vigorous agitation. The strains were grown in 1.5 l batches in 5 l Erlenmeyer flasks. For XL1-Blue(pGST-A1) and XL1-Blue(pGST-A2), expression was induced after reaching an OD (600 nm) of 0.7 by addition of isopropyl-β-d-thiogalactopyranoside (IPTG) at 0.75 mM and incubation was continued for about 18 h. The cells were harvested (5000 g, 20 min, 4°C) and washed with 20 mM PBS buffer (140 mM NaCl, 2.7 mM KCl, 10 mM Na2HPO4, 1.8 mM KH2PO4) before lysis. A freeze–thaw/sonication method was used for crude extract preparation. For this purpose, cells from 3 l of culture volume (about 9–10 g wet cell mass) were suspended in 2 ml of PBS buffer per gram of wet cell mass and subjected to three freeze(−20°C)–thaw(37°C) cycles. Lysis was completed by use of a UP200s sonicator (Dr Hielscher GmbH) with permanent cooling in an ice water slurry. Cellular debris was removed by centrifugation (40 000 g, 30 min, 4°C). The same method of crude extract preparation was used for XL1-Blue(pGST-A1A2), except that induction of expression was started with 1 mM IPTG at OD0.8, and the buffer for washing and lysis was 20 mM Tris/HCl pH 8. Typically, GST activities in the cleared crude extracts amounted to about 12 U mg−1 protein for XL1-Blue(pGST-A1A2) or 23–28 U mg−1 protein for XL1-Blue (pGST-A1) and XL1-Blue(pGST-A2). The GST hybrid proteins were purified via affinity chromatography on glutathione Sepharose 4B (Amersham-Pharmacia), an agarose gel matrix linked to epoxy-activated glutathione via a 10-carbon linker. For this purpose, a XK 16/10 column (Amersham-Pharmacia) packed with 10 ml of glutathione Sepharose 4B was equilibrated with PBS buffer. A sample of cleared crude extract (about 100 mg per run) prepared as described above was applied, thereafter the column was washed with PBS until the signal of the connected UV recorder returned to the base line. Specifically bound protein was eluted with a solution of 10 mM reduced glutathione in 50 mM Tris/HCl pH 8 at a flow rate of 2 ml min−1 while collecting fractions of 4 ml. The fractions were tested for GST activity and those with GST activity were analysed with SDS–PAGE. The following method was developed for the detection of polysaccharide binding by the XynA N-terminal domains and for the determination of their binding specificity. For this purpose, an electrophoretic technique described by 30 was modified to fit our needs. Then, 7.5% resolving gels for native polyacrylamide gel electrophoresis (native PAGE) were prepared by mixing 1 ml of 4 × resolving gel buffer (1.5 M Tris-HCl pH 8) with 1.8 ml of double distilled water, 1 ml of premixed 30% acrylamide/N,N′-methylenebisacrylamide (37.5:1) solution, 5 µl of N,N,N′,N′-tertamethylethylenediamine, and 60 µl of 10% (w/v) ammonium persulphate, and filling the solution between the glass plates of a Mini-Protean-II electrophoresis system (Bio-Rad). For native affinity PAGE (NAPAGE), soluble polysaccharides (see Table 1) were included in the resolving gel solution at a final concentration of 0.1%. The solution was carefully overlaid with water which was removed again after polymerization. The stacking gel (3%) was prepared on top of the resolving gel and was used for well formation by insertion of a Teflon comb. Protein samples were mixed with 0.33 volumes of 4 × sample buffer (0.5 M Tris-HCl pH 6.8–50% glycerol, 1% bromphenol blue) before application to the gel. The electrode chambers were filled with 25 mM Tris-HCl, 192 mM glycine pH 8.8 and electrophoresis was carried out at 80 V, followed by staining of the gel with Coomassie brilliant blue R-250. Determination of Kd values for polysaccharides was carried by performing NAPAGE at various concentrations of the ligand (30). Oat spelts xylan was covalently coupled to a divinylsulphone-activated agarose gel matrix (Mini-Leak High, Kem-En-Tec). Oat spelts xylan (5 g) was dissolved in 150 ml of 1 M sodium carbonate buffer pH 11 by autoclaving for 10 min. Insoluble material was removed by centrifugation (10.000 g, 15 min). Next, 30 ml of Mini-Leak High gel was washed and suspended in the alkaline xylan solution. The coupling reaction was allowed to proceed for 24 h at 45°C with weak agitation. The gel matrix was washed and suspended in bidistilled water (= immobilised xylan), degassed and packed into a XK16/10 column (100 mm × 16 mm) (Amersham-Pharmacia). The column was equilibrated with 10 mM Bis-Tris, 1 M NaCl pH 6.2 before application of protein samples dissolved in the same buffer. The loaded column was incubated for 2 h at room temperature, thereafter non-bound protein was washed from the column with equilibration buffer. Elution was achieved with a 0.5% solution of oat spelts xylan in bidistilled water at a flow rate of 2 ml min−1. Samples of the fractions collected were subjected to SDS–PAGE. Oat spelts xylan immobilized by covalent coupling to Mini-Leak High prepared as described above was used as an insoluble binding substrate for characterization of the binding properties of GST-A1A2. Standard binding assays (1 ml) were carried out in 1.5 ml plastic screw-cap vials. They contained 0.5 ml of 2 × binding buffer (20 mM Bis-Tris pH 6.2, 1 M NaCl), 0.2 ml of immobilised xylan suspension (50% slurry) and 1 U of GST fusion protein. The vials were incubated with slow rotation for 1.5 h at 20°C. The binding substrate was pelleted by centrifugation (5 min, 13.000 r.p.m. in a table-top centrifuge) and the supernatants were tested for GST activity or protein content. Each experiment included controls without binding substrate and without enzyme, and a control with 1 U of GST instead of fusion enzyme (to account for non-specific protein binding). Typically, more than 90% of the GST-A1A2 fusion protein bound to the immobilized xylan under these conditions. For determination of the effect of the pH on xylan binding, the standard buffer (0.5 ml of 20 mM Bis-Tris pH 6.2, 1 M NaCl in a 1 ml assay) was replaced with McIlvaine buffer (pH 3.0–8.0; 265 µl of a solution prepared by titration of 0.1 M citric acid and 0.2 M Na2HPO4), AMPSO (pH 8.5–9.5; 265 µl of a 150 mM stock solution) or CAPS (pH 10.0–11.0; 265 µl of a 150 mM stock solution), in each case supplemented with 0.5 M NaCl. Because GST is inactive at extreme pH values the protein content instead of GST activity was determined in the supernatant, using the Protein Assay Kit (microassay; Bio-Rad). For each pH tested a reference without binding substrate was measured. The protein content of this sample was defined as 100%. The influence of the NaCl concentration on the xylan-binding properties was tested by varying the NaCl concentration in the standard assay mixture between 0 M and 3 M. For each NaCl concentration investigated a reference without binding substrate was measured. The GST activity determined in this sample was defined as 100%. The binding of GST-A1A2 to xylan at various temperatures (4°C, 24°C, 30°C, 40°C, 50°C, 60°C, 70°C and 80°C) was investigated by incubation of the fusion protein under standard assay conditions in a hybridization oven. Because GST is inactive at extreme temperatures the protein content instead of GST activity was determined in the supernatant with the Protein Assay Kit (microassay; Bio-Rad). For each temperature tested a reference without binding substrate was measured. The protein content of this sample was defined as 100%. In order to determine the kinetics of polysaccharide binding of GST-A1A2 the fusion protein was incubated with immobilized xylan under the standard assay conditions for 0–120 min at room temperature. Samples incubated under the same conditions but without binding substrate served as references. In contrast to the standard binding assay, the centrifugation period for sedimentation of the binding substrate was shortened to 2 min. The influence of various mono-, di- and soluble polysaccharides on the binding of GST-A1A2 was investigated by adding 200 µl of the saccharide (monosaccharides, 1 M; disaccharides, 0.5 M; methyl cellulose, 1%; arabinogalactan, mannan, 2%; soluble fraction of 2% oat spelts xylan; soluble fraction of 5% oat spelts xylan before and after hydrolysis with 40 U ml−1 XynA at 75°C; soluble fraction of 5% Rhodymenia xylan) to the standard binding assay. For each saccharide two references were also tested; one without binding substrate (0% binding) and one without saccharide (100% binding). Binding of oligosaccharides to GST-A1A2 was measured with fluorescence titration. For this purpose, the protein concentration was determined by UV absorption in an Uvikon 931 spectrophotometer (Kontron). The specific extinction coefficient of GST-A1A2 was determined from the amino acid sequence according to 34 to 1.82 cm−1 mg−1 ml. Titration of XBD was performed in a stirred cuvette using a Fluoromax 2 spectrofluorimeter (Instruments S.A.) with a thermostated cuvette holder. Next, 3 µl of different stock solutions (pH 6.4) were added to 1.2 ml of a 0.22 µM GST-A1A2 solution (50 mM sodium phosphate, 100 mM NaCl, pH 6.4). After each addition, the sample was stirred for about 2 min. Fluorescence emission spectra were measured at an excitation wavelength of 280 nm with 3 nm excitation and 8 nm emission slit width. Maxima of the spectra as well as the respective signals at 366 nm (corresponding to the maximal difference of the fluorescence emission spectra between XBD and the XBD-saccharide complex) were determined. The signals were corrected for dilution and buffer effects. Because a fusion protein with GST was used, sugars were added to purified GST as a control. No signal changes and thus no binding was observed with this GST control. Assuming one binding site per monomer, the dissociation constant for saccharide binding was determined by non-linear least-squares fitting of the data to the equation with F, measured fluorescence; F0, fluorescence of the free protein; ΔF, change of fluorescence at saturation; P0 and L0, total concentrations of protein and saccharide; Kd, dissociation constant. Fits were obtained by using SigmaPlot (Jandel Scientific). The authors thank U. Ludwig for skilful technical assistance. Financial support by the Deutsche Forschungsgemeinschaft is gratefully acknowledged. Dr J. Puls, Hamburg and Dr W. Ludwig, München, are thanked for generous gifts of polysaccharide samples.Smith, J.A., etal. (1987) Current Protocols in Molecular Biology. New York: John Wiley and Sons.Laurie, J.I., Gilbert, H.J. (1995) A modular xylanase containing a novel non-catalytic xylan-specific binding domain. Biochem J 307: 191– 195.Morris, P., etal. (1996) Evidence that linker sequences and cellulose binding domains enhance the activity of hemicellulases against complex substrates. Biochem J 319: 515– 520.Bolam, D.N., etal. (1997) Cellulose binding domains and linker sequences potentiate the activity of hemicellulases against complex substrates. J Biotechnol 57: 59– 69.Kilburn, D.G., Warren, R.A.J. (1996) Probing the role of tryptophan residues in a cellulose-binding domain by chemical modification. Prot Sci 5: 2311– 2318.White, B.A., Mackie, R.I. (1999) Molecular cloning, sequencing, and expression of a novel multidomain mannanase gene from Thermoanaerobacterium polysaccharolyticum. J Bacteriol 181: 1643– 1651.Fontes, C.M.G.A., Hazlewood, G.P. (1996) A modular xylanase from mesophilic Cellulomonas fimi contains the same cellulose-binding and thermostabilising domains as xylanases from thermophilic bacteria. FEMS Microbiol Lett 139: 27– 35. Coughlan, M.P. & Hazlewood, G.P. (1993) β-1,4-d-xylan-degrading enzyme systems: biochemistry, molecular biology and applications. Biotechnol Appl Biochem 17: 259– 289.Kilburn, D.G., Miller, R.C. Jr (1992) The binding of Cellulomonas fimi endoglucanase C (CenC) to cellulose and Sephadex is mediated by the N-terminal repeats. Mol Microbiol 6: 1243– 1252.Haeberli, P., Smithies, O. (1984) A comprehensive set of sequence analysis programs for the VAX. Nucleic Acids Res 12: 387– 395.Warren, R.A.J., Kilburn, D.G. (1991) Non-hydrolytic disruption of cellulose fibres by the binding domain of a bacterial cellulase. Biotechnology 9: 1096– 1099.Warren, R.A.J., etal. (1994) The cellulose-binding domain of endoglucanase A (CenA) from Cellulomonas fimi: evidence for the involvement of tryptophan residues in binding. Mol Microbiol 11: 747– 755.Morosoli, R., Kluepfel, D. (1998) Substrate-binding domains of glycanases from Streptomyces lividans: characterization of a new family of xylan-binding domains. Biochem J 330: 41– 45.Hirst, B.A., Gilbert, H.J. (1995) Evidence for a general role for non-catalytic thermostabilizing domains in xylanases from thermophilic bacteria. Biochem J 307: 151– 158.Miller, R.C. Jr, Warren, R.A.J. (1991) Domains in microbial β-1,4-glycanases: sequence conservation, function and enzyme families. Microbiol Rev 55: 305– 315. Henrissat, B. & Davies, G. (1997) Structural and sequence-based classification of glycoside hydrolases. Curr Opin Struct Biol 7: 637– 644. Higgins, D.G. & Sharp, P.M. (1989) Fast and sensitive multiple sequence alignment on a microcomputer. CABIOS 5: 151– 153.Sleytr, U.B., etal. (1986) T. maritima sp. nov. represents a new genus of unique extremely thermophilic eubacteria growing up to 90°C. Arch Microbiol 144: 324– 333.Beaucamp, N., Ostendorp, R. (1996) Structure and stability of hyperstable proteins: glycolytic enzymes from the hyperthermophilic bacterium Thermotoga maritima. Adv Prot Chem 48: 181– 269.Schlegel, H.G. (1996) Biodegradation of polyhydroxyalcanoic acids. Appl Microbiol Biotechnol 46: 451– 463.Joshi, M.D., McIntosh, L.P. (1996) Interaction of polysaccharides with the N-terminal cellulose-binding domain of Cellulomonas fimi CenC. 2. NMR and ultraviolet absorption spectroscopy. Biochemistry 35: 13895– 13906.Rao, M. (1999) Molecular and biotechnological aspects of xylanases. FEMS Microbiol Rev 23: 411– 456.Lowe, S.E., Zeikus, J.G. (1993) Gene Cloning, Sequencing, and Biochemical Characterization of Endoxylanase from Thermoanaerobacterium saccharolyticum B6A-RI. Appl Environ Microbiol 59: 3134– 3137.Kellermann, J., Schleifer, K.H. (1992) Purification and characterization of a novel thermostable 4-α-glucanotransferase of Thermotoga maritima cloned in Escherichia coli. Eur J Biochem 207: 81– 88. Lindner, M. & Teeri, T.T. (1997) The roles and function of cellulose-binding domains. J Biotechnol 57: 15– 28.Clarke, J.H., Gilbert, H.J. (1994) Evidence for a general role for high-affinity non-catalytic cellulose binding domains in microbial plant cell wall hydrolases. Mol Microbiol 11: 375– 382.Nakamura, K., Takeo, K. (1992) Analysis of the interaction between an alpha (1→6) dextran-specific mouse hybridoma antibody and dextran B512 by affinity electrophoresis. J Chromatogr 597: 345– 350.Thomas, J., Bergquist, P.L. (1999) Family 10 and 11 xylanase genes from Caldicellulosiruptor sp. strain Rt69B.1. Extremophiles 3: 103– 111.Haft, D.H. etal. (1999) Evidence for lateral gene transfer between archaea and bacteria from genome sequence of Thermotoga maritima. Nature 399: 323– 329.Warren, R.A.J., Kilburn, D.G. (1993) The cellulose-binding domain (CBDcex) of an endoglucanase of Cellulomonas fimi: production in Escherichia coli and characterization of the polypeptide. Biotechnol Bioeng 42: 401– 409.Grimsley, G., Gray, T. (1995) How to measure and predict the molar absorption coefficient of a protein. Prot Sci 4: 2411– 2423.Virden, R., Gilbert, H.J. (1993) The role of conserved tryptophan residues in the interaction of a bacterial cellulose binding domain with its ligand. FEMS Microbiol Lett 106: 77– 84. Quiocho, F. (1993) Probing the interactions between proteins and carbohydrates. Biochem Soc Trans 21: 442– 448.Winterhalter, C., Liebl, W. (1997) Isolation and analysis of a gene encoding α-glucuronidase, an enzyme with a novel primary structure involved in the breakdown of xylan. Mol Microbiol 23: 267– 279.Ohmiya, K., Shimada, K. (1993) Nucleotide sequence of the Clostridium stercorarium xynA gene encoding xylanase A: Identification of catalytic and cellulose binding domains. Biosci Biotech Biochem 57: 273– 277.Fritsch, E.F., Maniatis, T. (1989) Molecular Cloning: A Laboratory Manual, 2nd edn. Cold Spring Harbor, NY: Cold Spring Harbor Laboratory Press. Schmid, F.X. (1997) Spectral methods of characterizing protein conformation and conformational changes. In Protein Structure: A Practical Approach. T.E. Creighton (ed. ). Oxford: IRL Press, pp. 261– 298.Johansson, G., Pettersson, G. (1991) A new model for enzymatic hydrolysis of cellulose based on the two-domain structure of cellobiohydrolase I. Biotechnology 9: 286– 290.Kimura, K., Ohmiya, K. (1998) Adsorption of Clostridium stercorarium xylanase A to insoluble xylan and the importance of the CBDs to xylan hydrolysis. J Ferm Bioeng 85: 63– 68.Kilburn, D.G., Haynes, C.A. (1996) Interaction of polysaccharides with the N-terminal cellulose-binding domain of Cellulomonas fimi CenC. 1. Binding specificity and Calorimetric analysis. Biochemistry 35: 13885– 13894.Shoham, Y., etal. (1996) Crystal structure of a bacterial family-III cellulose-binding domain: a general mechanism for attachment to cellulose. EMBO J 15: 5739– 5751. Vyas, N. (1991) Atomic features of protein–carbohydrate interactions. Curr Opin Struct Biol 1: 732– 740. Winterhalter, C. & Liebl, W. (1995) Two extremely thermostable xylanases of the hyperthermophilic bacterium Thermotoga maritima MSB8. Appl Environ Microbiol 61: 1810– 1815.Wich, G., Liebl, W. (1995) Identification of a novel cellulose-binding domain within the multidomain 120 kDa xylanase of the hyperthermophile bacterium Thermotoga maritima. Mol Microbiol 15: 431– 444.Vieira, J., Messing, J. (1985) Improved M13 phage cloning vectors and host strains: nucleotide sequence of the M13mp18 and pUC19 vectors. Gene 33: 103– 119.Velikodvorskaya, G., Borriss, R. (1996) The multidomain xylanase of the hyperthermophilic bacterium Thermotoga neapolitana is extremely thermoresistant. Appl Microbiol Biotechnol 45: 245– 247. The full text of this article hosted at iucr.org is unavailable due to technical difficulties. Please check your email for instructions on resetting your password. If you do not receive an email within 10 minutes, your email address may not be registered, and you may need to create a new Wiley Online Library account. Can\'t sign in? Forgot your username? Enter your email address below and we will send you your username If the address matches an existing account you will receive an email with instructions to retrieve your username
本文链接: https://www.ebiomall.com/b8-thermo/info-23434.html
免责声明 本文仅代表作者个人观点,与本网无关。其创作性以及文中陈述文字和内容未经本站证实,对本文以及其中全部或者部分内容、文字的真实性、完整性、及时性本站不做任何保证或承诺,请读者仅作参考,并请自行核实相关内容。
版权声明 未经蚂蚁淘授权不得转载、摘编或利用其他方式使用上述作品。已经经本网授权使用作品的,应该授权范围内使用,并注明“来源:蚂蚁淘”。违反上述声明者,本网将追究其相关法律责任。
相关文章
1970-01-01
1970-01-02
2005-01-01
1970-01-01
2008-05-26
2020-06-23
2008-12-20
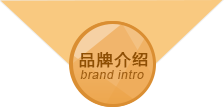
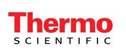
▍
品牌分类
▍
品牌问答
暂无品牌问答