购物车 |牛津生物医学研究所,Fluorescent dye
该抗体是在兔体内针对微粒体人 PGE 合酶(PGE 异构酶或 mPGEi)特异性合成肽生成的。 mPGEi 在体内炎症反应期间共同诱导(与 COX-2)PGH2 异构化为 PGE2。 mPGEi 是生理学和病理生理学研究的有用靶标。该抗体以血清形式提供,适用于 Westerns,尚未经过 IHC 测试。
Product DocumentsSpec Sheet PG15 SpecRelated ProductsProductCAT.#SizePriceAnti-Myeloperoxidase, Human Neutrophil, Rabbit IgG FractionGP501.0 ml$495.00CypExpress 2D6, 1.0 GramCE2D6.11.0克 500.00 克 CypExpress 19A1,1 克 CE19A1.1g1.0 克 465.00 克 CypExpress 1A1,10.0 克 CE1A1.1010.0 克 3,760.00 异前列烷 EIA 试剂盒 EA84 试剂盒 350.00 美元返回页首抗 PGD 合酶造血形式多克隆抗体 $290.00SKUPD01 产品概述该抗体是在兔体内制备的,使用大鼠造血形式 PGD 合酶,在 S-己基谷胱甘肽亲和树脂上纯化,作为免疫原。它对造血形式的 PGD 合酶具有特异性,不会与脑形式的 PGD 合酶发生交叉反应。该抗体可用于蛋白质免疫分析、免疫组织化学、免疫沉淀和 ELISA 应用。
背景前列腺素 D2 (PGD2) 在多种组织中活跃形成,并参与许多生理事件。 PGD2 是中枢神经系统 (CNS) 中主要的前列腺素之一,参与中枢神经系统活动,包括诱导体温过低和睡眠,被认为是一种新型神经调节剂。在哺乳动物的中枢神经系统和其他组织中,PGH2 异构化为 PGD2 是由 PGD 合酶催化的。已经报道了两种类型的PGD合酶。一种是需要谷胱甘肽 (GSH) 的 PGD 合成酶;另一个r 是不依赖于 GSH 的 PGD 合酶。这两种酶是非常相似的酸性蛋白质,具有相同的分子量,但区别在于它们在氨基酸组成、GSH 需求、对 1-氯-2,4-二硝基苯的敏感性和抗原决定簇方面不同。
Product DocumentsSpec Sheet PD01.pdfRelated ProductsProductCAT.#SizePriceActive Rat Urokinase, HMWVA570.05 mg$390.00CUPRACFood and Beverage Antioxidant AssayFS021 Kit$395.00GSH/GSSG Assay: CuvetteGT35Kit$385.00抗髓过氧化物酶、人中性粒细胞、兔 IgG分数GP501.0 毫升$495.00 CypExpress 2D6, 1.0 克 CE2D6.11.0 克 $500.00Fluorescent dyes possess reactive linkers for conjugating to nucleic acids, carbohydrates and peptides. The conjugates fluoresce in the visible and UV spectrum and have an excellant solvochromatic response as compared to other fluorescence or chromatic labels. The conjugates are stable but also have medium sensitive. The fluorescent dyes have little triplet state formation and are not photoreactive, making them an excellent substance for biological investigations. Uses for the dyes include protein labelling, DNA labelling, single molecule spectroscopy and fluorescence. A synthesis of the dyes is disclosed. Methods of use include the detection of carbohydrate-protein interactions. 435/6.13, 435/7.72, 435/7.8, 435/14, 435/23, 435/24, 435/968, 536/17.2, 536/17.3, 536/17.7, 536/18.7 435/4, 435/968, 435/6, 435/7.72, 435/7.8, 435/14, 435/23, 435/24, 536/17.2, 536/17.3, 536/17.7, 536/18.7 What is claimed is: 1. A fluorescent dye represented by the following structure: ##STR28## wherein R1 is selected from the group consisting of radicals represented by the following structures: ##STR29## wherein R2 and R3 are independently selected from a group consisting of hydrogen and radicals represented by the following structures: ##STR30## wherein 1≤n≤4; and wherein R4 and R5 are independently selected from the group consisting of C1-C6 alkyls. 2. The fluorescent dye as described in claim 1 represented by the following structure: ##STR31##. 3. The fluorescent dye as described in claim 1 represented by the following structure:. 4. The fluorescent dye as described in claim 1 represented by the following structure:. 5. The fluorescent dye as described in claim 1 represented by the following structure: wherein R is selected from a group consisting of ##STR32##. 6. A fluorescent conjugate represented by the following structure: D--L--Bwherein:D-- is a fluorescent dye having a radical portion and is represented by the following structure: ##STR33## wherein R1 is selected from the group consisting of radicals represented by the following structures: ##STR34## wherein R4 and R5 are independently selected from the group consisting of C1-C6 alkyls and R2 and R3 are independently either hydrogen or absent so as to form a radical;with a proviso that one of R2 and R3 is absent so as to form the radical of said fluorescent dye;--L-- is a diradical linker selected from a group represented by the following structures: ##STR35## wherein 1≤n≤4; and --B is a biomolecule having a radical portion, wherein the radical portion is selected from a group consisting of hydroxyl radical and amino radical;whereinsaid diradical linker --L-- links the radical of said fluorescent dye D-- to the radical of said biomolecule --B to form said fluorescent conjugate. 7. The fluorescent conjugate as described in claim 6 wherein said biomolecule is selected from a group consisting of carbohydrate, nucleic acid, and peptide. 8. The fluorescent conjugate as described in claim 7 represented by the following structure: ##STR36##. 9. The fluorescent conjugate as described in claim 7 represented by the following structure:. 10. A fluorescent conjugate represented by the following structure: D--Bwherein:D-- is a radical of a fluorescent dye represented by the following structure: ##STR37## wherein R4 and R5 are independently selected from the group consisting of C1-C6 alkyls; and--B is a biomolecule having a radical portion, wherein the radical portion is selected from a group consisting of hydroxyl radical and amino radical;whereinthe radical of said fluorescent dye D-- is linked to the radical portion of said biomolecule --B to form said fluorescent conjugate. 11. The fluorescent conjugate as described in claim 10 wherein said biomolecule is selected from a group consisting of carbohydrate, nucleic acid, and peptide. 12. A fluorescent conjugate represented by the following structure: B1 --L--D--L--B2wherein:D-- is a fluorescent dye having two radicals portions and is represented by the following structure: ##STR38## --L-- is a diradical linker selected from a group represented by the following structures: ##STR39## wherein 1≤n≤4; and B1 -- and --B2 are independently selected from a group of biomolecules, each biomolecule having a radical portion, wherein the radical portion is selected from a group consisting of hydroxyl radical and amino radical;whereinsaid diradical linker --L-- links each of the radicals of said fluorescent dye D-- to the radical of each of said biomolecules B1 -- and --B2 to form said fluorescent conjugate. 13. The fluorescent conjugate as described in claim 12 wherein each of said biomolecules B1 -- and --B2 are independently selected from a group consisting of carbohydrate, nucleic acid, and peptide. 14. A fluorescent conjugate represented by the following structure: B1 --D--L--B2wherein:D-- is a fluorescent dye having a hydroxyl radical and an amino radical and is represented by the following structure: ##STR40## --L-- is a diradical linker selected from a group represented by the following structures: ##STR41## wherein 1≤n≤4; and B1 -- and --B2 are independently selected from a group of biomolecules, each biomolecule having a radical portion, wherein the radical portion is selected from a group consisting of hydroxyl radical and amino radical;whereinsaid diradical linker --L-- links the amino radical of said fluorescent dye D-- to the radical of said biomolecule --B2 and the hydroxyl radical of said fluorescent dye D-- is linked directed to the radical of said biomolecule --B1. 15. The fluorescent conjugate as described in claim 14 wherein each of said biomolecules B1 -- and --B2 are independently selected from a group consisting of carbohydrate, nucleic acid, and peptide. 16. A fluorescent conjugate represented by the following structure: D1 --L1 --B--L2 --D2wherein:D1 and D2 are each a fluorescent dye having a radical portion and are each independently represented by the following structure: ##STR42## wherein R1 is independently selected from the group consisting of radicals represented by the following structures: ##STR43## wherein R4 and R5 are independently selected from the group consisting of C1-C6 alkyls and R2 and R3 are independently either hydrogen or absent so as to form a radical;with a proviso that, within each of said D1 and D2, one of R2 and R3 is absent so as to form the radical of said fluorescent dye;L1 and L2 are each diradical linkers independently selected from a group represented by the following structures: ##STR44## wherein 1≤n≤4; and --B is selected from a group of biomolecules, each biomolecule having a first and a second radical portion, wherein the first and second radical portions are independently selected from a group consisting of hydroxyl radical and amino radical;whereinsaid fluorescent dye D1 is linked to the first radical of said biomolecule by said diradical linker --L--; andsaid fluorescent dye D2 is linked to the second radical of said biomolecule by said diradical linker --L-- to form said fluorescent conjugate. 17. The fluorescent conjugate as described in claim 16 wherein said biomolecule B-- is selected from a group consisting of carbohydrate, nucleic acid, and peptide. 18. A fluorescent conjugate represented by the following structure: D1 --B--L2 --D2wherein:D1 is a radical of a fluorescent dye represented by the following structure: ##STR45## wherein R4 and R5 are independently selected from the group consisting of C1-C6 alkyls;D2 is a fluorescent dye having a radical portion represented by the following structure: ##STR46## wherein R1 is independently selected from the group consisting of radicals represented by the following structures: ##STR47## wherein R2 and R3 are independently either hydrogen or absent so as to form a radical;with a proviso that, within each of said D1 and D2, one of R2 and R3 is absent so as to form the radical of said fluorescent dye;L is a diradical linker selected from a group represented by the following structures: ##STR48## wherein 1≤n≤4; and --B is selected from a group of biomolecules, each biomolecule having a first and a second radical portion, wherein the first and second radical portions are independently selected from a group consisting of hydroxyl radical and amino radical;whereinsaid fluorescent dye D1 is linked directly to the first radical of said biomolecule; andsaid fluorescent dye D2 is linked to the second radical of said biomolecule by said diradical linker --L-- to form said fluorescent conjugate. 19. The fluorescent conjugate as described in claim 18 wherein said biomolecule B-- is selected from a group consisting of carbohydrate, nucleic acid, and peptide. 20. A method for identifying an interaction between a dye labeled biomolecule and a binding molecule wherein the method comprises the following steps:Step A: providing a dye labeled biomolecule selected from a group consisting of one of the following structures: ##STR49## wherein R1 and R2 are each independently selected from a group consisting of hydrogen, a carbohydrate, a nucleic acid, a peptide and a radical represented by one of the following structures: ##STR50## wherein R3 and R4 are each independently selected from a group consisting of hydrogen, a carbohydrate, a nucleic acid and a peptide; 1≤n≤4; for forming a dye labeled biomolecule; thenStep B: admixing the dye labeled biomolecule with a binding molecule; and thenStep C: selectively detecting a binding event with the dye labeled biomolecule described in Step B and the binding molecule. 21. A method for synthesizing a dye molecule represented by the following structure: ##STR51## wherein R5 is selected from a group consisting of one of the following structures: ##STR52## the method comprising the following steps: Step A: providing an aldehyde molecule represented by the following structure: ##STR53## Step B: condensing the aldehyde molecule of said Step A with a phosphodiester represented by the following structure: ##STR54## for making the dye molecule. TECHNICAL FIELDThe present invention relates to a new class of solvent matrix sensitive dyes which possess reactive linkers for attachment to nucleic acids, carbohydrates and peptides. These derivatives operate in the visible and UV spectrum. In particular, uses for the dyes include protein labelling, DNA labelling, single molecule spectroscopy and fluorescence. BACKGROUND Within the last decade, the development of improved electron tunneling probes and optical methods has made it possible to detect single molecules in vacuum, solution, the solid-state and on surfaces. In contrast to classical spectroscopy which monitors an average molecular ensemble, single molecule detection (SMD) examines unique events within a population. This ability enables one to examine minor species whose function would normally be lost by inclusion within an average. In solution, single molecules have been detected by monitoring their laser-induced fluorescence (LIF) (Rigler, R. J. Biotech. 1995, 41, 177; Rigler et al. Fluorescence Spectroscopy; Wolfbeis O. S., Ed.; Springer, Berlin, 1992, pp 13-24; Edman et al. Proc. Natl. Acad. Sci. USA 1996, 93, 6710; Goodwin et al. Acc. Chem. Res. 1996, 29, 607; Schmidt et al. Proc. Natl. Acad. Sci. USA 1996, 93, 2926; Keller et al. Appl. Spectroscopy 1996, 50, A12). While the detection limit of LIF is far superior to that of traditional fluorescence spectroscopy, the method cannot directly operate at the single molecule level (i.e., 10-24 M). Amplification to the molecular level can be accomplished by reducing the probed volume to or below a femtoliter. Modern developments in optical imaging now provide confocally-imaged pin holes which when placed in the path of a diffraction limited laser beam provide an illuminated cylinder with a diameter of 400 nm, a length of 2 mm, and a volume of approximately 0.2 fl (1 fl=10-15 1). In this cavity, the concentration of a single molecule now corresponds to 8.3 nM, a value within the capacity of LIF. Using this and other methods for generation of small volumes, single fluorescent molecules have been detected in flowing and static solutions. Since its discovery in 1974, fluorescence correlation spectroscopy (FCS) has provided new insight into a wide variety of investigations; including diffusion, aggregation, chemical reactions, and conformational analysis. The principle of this method is based on monitoring the fluctuation in fluorescence intensity as molecules diffuse through a specified illuminated cavity. When a molecule or group of molecules pass into a cavity tuned near their absorption maximum, they undergo cycles of excitation and relaxation by emission of a second photon. When these events are recorded in a time-dependent matter, the quanta which belongs to these molecules can be determined through autocorrelation. In 1994, Rigler and Eigen described a method for detecting single rhodamine-labelled DNA molecules, based application of confocal microscopy to FCS. Concurrently, Zare and colleagues devised a similar method for detecting single molecules of YOYO intercalated DNA in real time, without the need for autocorrelation. Since these discoveries, Webb and Gratton have expanded the spectral window to include the UV-region through use of multiple photon excitation. When used in conjunction with techniques for concentrating or separating particles, such as electrical focusing or optical tweezers, the detection limit of this method becomes infinite. To date, FCS has been applied to a number of biophysical investigations, including the study of protein folding, neuroreceptor-binding, the motion of actin-filaments, and membrane dynamics. This investigation describes the first application of single molecule FCS to monitor the interaction between carbohydrates and proteins. Recently, increased attention has been devoted to gaining a better understanding of the biological significance of carbohydrate-protein recognition, due to the participation of these events in a wide variety of disease related processes including: cellular growth-development, fertilization, metastasis, inflammatory response, as well as bacterial and viral recognition. Low affinity, often with an association constant (Ka) as low as 10-4 M-1, has been a major problem facing these investigations. One solution to this problem has appeared through enhancement of the binding by modification of one partner. Several groups have reported dramatically increased affinities of molecules possessing multiple (polyvalent) carbohydrates. The affinity of these ligands and their monomeric counterparts can be determined with techniques such as fluorescence anisotropy or microcalorimetry. The advantage of the latter is that it provides a complete energetic description, including entropic and enthalpic terms. These method have been used to determine the affinity of sialosides for influenza hemagglutinin, various oligosaccharides for E-selectin, Salmonella trisaccharide epitope for a monoclonal antibody Se 155-4, and numerous C- and O-glycosides for lectins. Although accurate, both methods rely on a comparison between the free and bound state. To date, neither method is capable of specifically detecting molecules in one state nor can either method operate at the single molecule level. One question that becomes important to the understanding of carbohydrate-protein binding events is the role and mechanistic aspects of aggregation before and after binding. Early on, it was recognized that several of the carbohydrate binding proteins exist in aggregated (dimeric, tetrameric, or polymeric) forms. Multivalent ligands, which are already polymeric, are biased towards aggregated forms and therefore do not easily allow one to examine this aggregation. Therefore, discovery of a method which selectively detects only the carbohydrate-bound or free state provides an ideal tool for this type of investigation. Based on the low affinities of these events, the design must incorporate a means to detect at very low concentration, ideally at the single molecule level. One limitation to the development of confocal FCS and further laser-based fluorometric techniques is the photophysical and spectroscopic properties of the fluorescent molecule or tag. In fluorescence-based single molecule detection, a laser beam tuned near the absorption maximum of the fluorophore is used to initially provide high-lying rotational and vibrational states which then undergo picosecond non-radiative decay to a low-lying singlet state (S1). In doing so, molecules which contain degrees of rotational freedom can adopt more than one singlet state, such as twisted intramolecular charge transfer (TICT) states. These states originate from internal rotation to conformers where the orbitals of one portion of the molecule are oriented orthogonally with the other. Observation of these states was first seen in the fluorescence spectrum of p-N,N-dimethylaminobenzonitrile and soon thereafter attributed internal twisting about the dimethylamino group through analogy to several locked and rotationally restricted derivatives, such as those shown in FIG. 1. Emission from these states is typically sensitive to solvent polarity, low in energy and intensity, and of short lifetime. In addition to formation of TICT states, several fluorophores readily undergo spin-forbidden relaxation from the S1 state to a long-lived triplet state (T1), additionally decreasing in their fluorescence. For single molecule detection, the efficiency of a chromophore is measured with its absorption cross-section (s), its fluorescence quantum yield (Ff) and its photodecomposition. In addition to photophysical considerations, fluorometric detection of binding requires a substance which undergoes significant modification of its absorption or emission maxima, emission quantum yield and/or excited state lifetime upon binding. Unlike many commonly used fluorophores, (these quantum yields can be compared to other commonly used fluorophores such as fluorescein (0.91 in 0.1 M NaOH) or Rhodamine B (0.70 in is ethanol). Chen, R. F. Anal. Biochem. 1967, 20, 339) the quantum yield of p-(N,N-dimethylamino)-p\'-nitro-trans-trans-1,4-diphenyl-1,3-butadiene (NND) decreases upon changing from non-polar to polar solvent systems as seen in the comparison of heptane (Ff=0.097) to methanol (Ff=4×10-6). 17 In addition, the absorption and fluorescence maximum of these materials is red shifted by a respective 130 and 109 nanometers over the same interval. Even more remarkably, the quantum yield of this material increased to 0.14, a 44% improvement over that seen in the most non-polar solvent, when embedded in a phospholipid vesicle. (Shin et al. J. Phys. Chem. 1988, 92, 2945). This fact clearly shows that restriction of the space for internal rotation results in a dramatic gain of fluorescence. Similar findings have been seen in the insertion of trans-stilbene into vesicle membranes (Suddabay, et al. J. Am. Chem. Soc. 1985, 107, 5609). In this case, the increased fluorescence quantum yield was attributed to inhibiting photoisomerization, to cis-stilbene, which is known to compete for the singlet excited state with fluorescence. Comparable isomerizations have been seen in p-(N,N-dimethylamino)-p\'-nitro-trans-1,2-diphenyl-1,3-ethylene (NNS) (Gorner et al. J. Mol. Struct. 1982, 84, 227; Bent et al. J. Phys. Chem. 1974, 78, 446; Gorner, H.; Schulte-Frohlinde, D. Ber. Bunsen-Ges. Phys. Chem. 1978, 82, 1102). The isomerization yield of this process (Ft-c=0.034 in toluene) as well as the amount of crossing to the triplet state decreased with solvent polarity, suggesting that non-radiative relaxation was the major path back to the ground state in polar solvents. When extensively photolyzed, NND in cyclohexane or toluene reached a photostationary state containing a mixture of the initial trans-trans-isomer (67%) and the corresponding cis-trans isomers. Inhibition of this isomerization may be one factor contributing to the increased fluorescence seen in vesicles. Alternatively, this fluorescence gain can be explained by restricting the formation of TICT states. Although there is no direct evidence for the presence of TICT states in NND, due to the complicated nature associated with several positions for rotation, the decreased fluorescence and red-shifted absorption upon increasing solvent polarity is comparable with substances known to have TICT states. Charge transfer labels, such as 5-(dimethylamino)-1-naphthalenesulfonyl or dansyl chloride, have been extensively used for the detection, characterization and localization of carbohydrates, phospholipids, proteins, oligonucleotides as well as numerous other synthetic and natural materials (Seiler et al. Biochem. Anal. 1970, 18, 259). These materials typically experience a shift in their UV/visible absorption and/or fluorescence spectra with respect to the nature of their solvent shell (Reichardt et al., Chem. Rev. 1994, 94, 2319; Kosower et al. J. Am. Chem. Soc. 1971, 93, 2713; Kamlet et al, J.-L. M. Abboud, R. W. Taft, ibid. 1977, 99, 8325). This effect as well as additional modification of their fluorescence lifetime, amount of intersystem crossing and fluorescence quantum yield have encouraged their use as practical sensors for monitoring the interactions of biologically relevant macromolecules (Weber et al. J. Biochem. 1954, 56, xxxi; Stryer, J. Mol. Biol. 1965, 13, 482; Gally et al. Biochim. Biophys. Acta 1965, 94, 175; Cory et al. J. Am. Chem. Soc. 1968, 90, 1643; Chen et al. Biochem. 1967, 120, 609; Guest et al. Biochem. 1991, 30, 8759). Given by the extent of aromaticity, the dansyl group absorbs light between =190 and 400 nm, limiting its excitation primarily to ultraviolet light (this can be circumvented through use of two or multi-photon excitation, see: Xu et al. J. Opt. Soc. Am. B, 1996, 13, 481). What is needed is a new class of intramolecular charge-transfer labels which absorb visible and ultraviolet light, display a dramatic solvent sensitivity and can be detected at the single molecule level. SUMMARY OF THE INVENTION The invention is directed to specific fluorescent dyes which have reactive linkers for conjugating to nucleic acids, carbohydrates and peptides. The conjugates fluoresce in the visible and UV spectrum and have an excellant solvochromatic response as compared to other fluorescence or chromatic labels. The conjugates are stable but also have medium sensitive. The fluorescent dyes have little triplet state formation and are not photoreactive, making them an excellent substance for biological investigations. Uses for the dyes include protein labelling, DNA labelling, single molecule spectroscopy and fluorescence. A synthesis of the dyes and of dye conjugates is disclosed. Methods of use include the detection of carbohydrate-protein interactions. One aspect of the invention is directed to a fluorescent dye represented by the following structure: ##STR1## R1 is selected from the group consisting of radicals represented by the following structures: ##STR2## R2 and R3 are independently selected from a group consisting of hydrogen and radicals represented by the following structures: ##STR3## wherein 1≤n≤4. R4 and R5 are independently selected from the group consisting of C1-C6 alkyls. Preferred embodiments of this aspect of the invention are fluorescent dyes represented by the following structures: ##STR4## wherein R is selected from a group consisting of ##STR5## Another aspect of the invention is directed to a fluorescent conjugate represented by D--L--B. D-- is a fluorescent dye having a radical portion and is represented by the following structure: ##STR6## R1 is selected from the group consisting of radicals represented by the following structures: ##STR7## R4 and R5 are independently selected from the group consisting of C1-C6 alkyls and R2 and R3 are independently either hydrogen or absent so as to form a radical, with a proviso that one of R2 and R3 is absent so as to form the radical of the fluorescent dye. --L-- is a diradical linker selected from a group represented by the following structures: ##STR8## wherein 1≤n≤4. --B is a biomolecule having a radical portion, wherein the radical portion is selected from a group consisting of hydroxyl radical and amino radical. The diradical linker --L-- links the radical of the fluorescent dye D-- to the radical of the biomolecule --B to form the fluorescent conjugate. In preferred embodiments, the biomolecule is selected from a group consisting of carbohydrate, nucleic acid, and peptide. Examples of preferred embodiment are represented by the following structures: ##STR9## Another aspect of the invention is directed to a fluorescent conjugate represented by D--B. D-- is a radical of a fluorescent dye represented by the following structure: ##STR10## R4 and R5 are independently selected from the group consisting of C1-C6 alkyls. --B is a biomolecule having a radical portion, wherein the radical portion is selected from a group consisting of hydroxyl radical and amino radical. The radical of the fluorescent dye D-- is linked to the radical portion of the biomolecule --B to form the fluorescent conjugate. In preferred embodiments, fluorescent conjugate the biomolecule is selected from a group consisting of carbohydrate, nucleic acid, and peptide. Another aspect of the invention is directed to a fluorescent conjugate represented by B1 --L--D--L--B2. D-- is a fluorescent dye having two radicals portions and is represented by the following structure: ##STR11## --L-- is a diradical linker selected from a group represented by the following structures: ##STR12## wherein 1≤n≤4. B1 -- and --B2 are independently selected from a group of biomolecules, each biomolecule having a radical portion, wherein the radical portion is selected from a group consisting of hydroxyl radical and amino radical. The diradical linker --L-- links each of the radicals of the fluorescent dye D-- to the radical of each of the biomolecules B1 -- and --B2 to form the fluorescent conjugate. In preferred embodiments, each of the biomolecules B1 -- and --B2 are independently selected from a group consisting of carbohydrate, nucleic acid, and peptide. Another aspect of the invention is directed to a fluorescent conjugate represented by B1 --D--L--B2. D-- is a fluorescent dye having a hydroxyl radical and an amino radical and is represented by the following structure: ##STR13## --L-- is a diradical linker selected from a group represented by the following structures: ##STR14## wherein 1≤n≤4. B1 -- and --B2 are independently selected from a group of biomolecules, each biomolecule having a radical portion, wherein the radical portion is selected from a group consisting of hydroxyl radical and amino radical. The diradical linker --L-- links the amino radical of the fluorescent dye D-- to the radical of the biomolecule --B2 and the hydroxyl radical of the fluorescent dye D-- is linked directed to the radical of the biomolecule --B1. In preferred embodiments, the biomolecules B1 -- and --B2 are independently selected from a group consisting of carbohydrate, nucleic acid, and peptide. Another aspect of the invention is directed to a fluorescent conjugate represented by D1 --L1 --B--L2 --D2. D1 and D2 are each a fluorescent dye having a radical portion and are each independently represented by the following structure: ##STR15## R1 is independently selected from the group consisting of radicals represented by the following structures: ##STR16## R4 and R5 are independently selected from the group consisting of C1-C6 alkyls. R2 and R3 are independently either hydrogen or absent so as to form a radical. However, there is a proviso that, within each of D1 and D2, one of R2 and R3 is absent so as to form the radical of the fluorescent dye. L1 and L2 are each diradical linkers independently selected from a group represented by the following structures: ##STR17## wherein 1≤n≤4. --B is selected from a group of biomolecules, each biomolecule having a first and a second radical portion, wherein the first and second radical portions are independently selected from a group consisting of hydroxyl radical and amino radical. The fluorescent dye D1 is linked to the first radical of the biomolecule by the diradical linker --L--. The fluorescent dye D2 is linked to the second radical of the biomolecule by the diradical linker --L-- to form the fluorescent conjugate. In preferred embodiments, the biomolecule B-- is selected from a group consisting of carbohydrate, nucleic acid, and peptide. Another aspect of the invention is directed to a fluorescent conjugate represented by D1 --B--L2 --D2. D1 is a radical of a fluorescent dye represented by the following structure: ##STR18## R4 and R5 are independently selected from the group consisting of C1-C6 alkyls. D2 is a fluorescent dye having a radical portion and is represented by the following structure: ##STR19## R1 is independently selected from the group consisting of radicals represented by the following structures: ##STR20## R2 and R3 are independently either hydrogen or absent so as to form a radical. However, there is a proviso that, within each of the D1 and D2, one of R2 and R3 is absent so as to form the radical of the fluorescent dye. L is a diradical linker selected from a group represented by the following structures: ##STR21## wherein 1≤n≤4. --B is selected from a group of biomolecules, each biomolecule having a first and a second radical portion, wherein the first and second radical portions are independently selected from a group consisting of hydroxyl radical and amino radical. The fluorescent dye D1 is linked directly to the first radical of the biomolecule; and the fluorescent dye D2 is linked to the second radical of the biomolecule by the diradical linker --L-- to form the fluorescent conjugate. In preferred embodiments, biomolecule B-- is selected from a group consisting of carbohydrate, nucleic acid, and peptide. Another aspect of the invention is directed to a method for identifying an interaction between a dye labeled biomolecule and a binding molecule. In the first step of the method, a dye labeled biomolecule is provided wherein the dye labeled biomolecule is selected from a group consisting of one of the following structures: ##STR22## R1 and R2 are each independently selected from a group consisting of hydrogen, a carbohydrate, a nucleic acid, a peptide and a radical represented by one of the following structures: ##STR23## R3 and R4 are each independently selected from a group consisting of hydrogen, a carbohydrate, a nucleic acid and a peptide; 1≤n≤4. Then, the dye labeled biomolecule is admixed with a binding molecule. And then, a binding event is selectively detected between the dye labeled biomolecule described above and the binding molecule. Another aspect of the invention is directed to a method for synthesizing a dye molecule represented by the following structure: ##STR24## R5 is selected from a group consisting of one of the following structures: ##STR25## In the first step of the method, an aldehyde molecule is provided wherein the aldehyde molecule is represented by the following structure: ##STR26## Then, the above aldehyde molecule is condensed with a phosphodiester represented by the following structure: ##STR27## The product is the desired dye molecule. BRIEF DESCRIPTION OF FIGURES FIG. 1 illustrates observation of states seen in the fluorescence spectrum of p-N,N-dimethylaminobenzonitrile and attributed internal twisting about the dimethylamino group through analogy to several locked and rotationally restricted derivatives. FIG. 2 illustrates the synthesis of two analogs of NND, compound 3 and compounds 4. Compound 4 is the title compound: 1- 4-(N,N-dimethylamino)-phenyl)-4-(3-hydroxy-4-nitrophenyl)-3-(E)-buten-1 -yne (DHNB). FIG. 3 shows the synthesis of 3 with the following steps: (a) NaBH4, MeOH, THF, Et2 O (5:5:1), 0° C. to rt, 97%. (b) CBr4, PPh3, CH2 Cl1, 0° C. to rt, 86%. (c) P(OEt)3, DMF, 155° C., 1.5 h, 99%. (d) i. add NaHMDS (2.2 eq) in THF to crude 8 in DMF, 0° C. to rt, 1 h; ii. add 9 in THF, -20° C. to rt, 6 h, 55%. FIG. 4 illustrates the synthesis of 1, 2 and 4 with the following steps: (a) i. iodine, NaHCO3, H2 O, 12-15° C., 10 min; ii. then rt, 1 h, 86%. (b) propargyl alcohol, Cl2 Pd(PPh3)2 (1.4 mol %), CuI (1.4 mol %), Et3 N, rt, 18 h, 89%. (b) MnO2, CH2 Cl2, rt, 4 h, 89%. (d) i. add NaHMDS (2.2 eq) in THF to crude 8 in DMF, 0° C. to rt, 1 h; ii. add 13 in THF, -20° C. to rt, 6 h, 56%. (e) i. α-D-maltopyranosylbromide heptaacetate, N-benzyltriethylammonium chloride, 1 M NaOH, CH2 Cl2, rt, 12 h; ii. NaOCH3, CH3 OH, rt, 1 h; iii. PhCO2 H; iv. powdered 4 Å mol. sieves, 1,4-dioxane, 70° C., 2 h; 69%. (f) i. α-D-glucopyranosylbromide tetraacetate, N-benzyltriethylammonium chloride, 1 M NaOH, CH2 Cl2, rt, 12 h; ii. NaOCH3, CH3 OH, Ph, rt, 1 h; iii. PhCO2 H; iv. powdered 4 Å mol sieves, 1,4-dioxane, 70° C., 2.5 h; 71%. FIG. 5 shows the complete scheme for the synthesis of 1, 2 and 4 wherein the reaction steps, depicted as arrows for each transition, are exactly as described in FIGS. 3 and 4. FIG. 6 shows a table of absorbtion maxima, extinction coefficient, fluorescence maximum and quantum yield of glycodyes 1 and 2. FIG. 7 illustrates the current design mimic strategy which incorporates 1- 4-(N,N-dimethylamino)-phenyl)-4-(3-hydroxy-4-nitrophenyl)-3-(E)-buten-1 -yne (DHNB) group, a water solubilizing piperizine linker, a fluorescence enhancer and a reactive linker component. FIG. 8 illustrates the synthesis of compound 900 with the following steps: (a) di-tert-butyl dicarbonate, Et3 N, DMAP, CH2 Cl2, 0° C. to rt, 94%. (b) 1) iodine, NaHCO3, CH2 Cl2, H2 O, 12-15° C., 10 min; 2) then rt, 30 min, 90%. (c) propargyl alcohol, Cl2 Pd(PPh3)2, CuI, Et3 N, THF, rt, 18 h, 85%. (d) MnO2, CH2 Cl2, rt, 6 h, 98%. (e) 1) add NaHMDS in THF to 800 in DMF, 0° C. to rt, 1 h; 2) add 700 in THF, -20° C. to rt, 8 h, 62%. FIG. 9 illustrates the synthesis of compound 100, 200, 300 and 1000 with the following steps: (a) 1) H2 SO4, H2 O, THF, rt; 2) glutaric anhydride, THF, rt, 2 h; 3) N-hydroxysuccinimide, EDC, THF, rt, 12 h, 81%. (b) 1) H2 SO4, H2 O, THF, rt; 2) glutaric anhydride, THF, rt, 2 h; iii. EDC, MeOH, THF, rt, 4 h, 77%. (c) N-hydroxysuccinimidyl glutaryl chloride, DMAP, THF, rt, 12 h, 64%. (d) N,N-carbonyldimidazole (2.5 eq), DMAP (0.05 eq), THF, rt, 14 h, 72%. FIG. 10 illustrates a table which shows UV/Visible Absorption Maxima, Range, Extinction Coefficients (ε), Fluorescence Maxima, Range and Quantum Yields (Φf) for Compound 1000 in Various Anhydrous Solvents with the following footnotes: a! Data provided is average over several iterations with a deviation within 5%. Although unavoidable, traces of moisture undoubtedly decreased the intensity of these values. Caution was taken to minimize contact with moisture and all solvents were either purchased dry and or distilled. b! See Reichardt, Chem. Rev. 1994, 94, 2319; c! The K-band is defined as the lower and the B-band as the higher wavelength band; d! Range is defined as the region in which 5% of the maximum absorption was apparent; e! The quantum yields were standardized against 0.70 for rhodamine B in ethanol. FIG. 11 illustrates single Molecule Detection of Compound 1000 with the following footnotes: a! Excitiation was provided with an argon ion laser at 455 nm (0.5 mW) and the fluorescence was filtered with a 545 nm cutoff filter; b! Detection limit is given as the concentration at which transients from single molecules were no longer resolved from the baseline. FIG. 12 illustrates real time and autocorrelated traces demonstrating carbohydrate binding to Con A. Samples were in buffer which was 10 mM in MnCl2, 10 mM in CaCl2, 0.05 M in PIPES (pH=7.2) and 1 M in NaCl, irradiated with a laser tuned to 457 nm (0.5 mW) and collected through an Omega Optics 545 cutoff filter. For each sample, the upper trace indicates the frequency of emitted photons (in kHz) and shows transients from aggregates in real time. The lower provides the autocorrelation function, where the number of particles inside the volume element is given by 1/g2 as t to 0. The midpoint of the autocorrelated curve provides an approximation of the diffusion time. (A) 51 mM 1, (B) 12 mM 2, (C) 51 mM 1 with 170 mM Con A and (D) 12 mM 2 with 170 mM Con A. FIG. 13 illustrates the behavior of compounds 1-4 in the Eigen-Rigler confocoal fluorescence correlation spectrometer wherein excitiation was provided with at 455 nm (0.5 mW) and the fluorescence was collected through a 545 nm cutoff filter. DETAILED DESCRIPTION OF THE INVENTION The invention is directed to the construction of a family of DHNB (1- 4-(N,N-dimethylamino)-phenyl)-4-(3-hydroxy-4-nitrophenyl)-3-(E)-buten- 1-yne (compound 4)) derivatives which can be used to label a wide variety of biologically-significant molecules. The labeling of molecules with charge-transfer dyes, such as 5-(dimethylamino)-1-napthalenesulfonyl (dansyl) chloride, is a powerful tool for examining the solvent shell of attached substances. The present invention involves the synthesis and application of a new charge transfer label, based on p-(N,N-dimethylamino)-p\'-nitro-trans-trans-1,4-diphenyl-1,3-butadiene (NND) (Shin et al. J. Phys. Chem. 1988, 92, 2945). Unlike many commonly used fluorophores, the quantum yield of NND decreases over four orders of magnitude upon changing from non-polar to polar environments. In addition, several derivatives of NND undergo little photodecomposition and can be detected at the picomolar level in a confocal fluorescence correlation spectrometer. In conjunction with recent detection of single molecules in solution, one aspect of the invention, as described in example 1, below, describes a method to discriminate between single free and carbohydrate-bound aggregates of the Jack Bean lectin, Concanavalin A (Con A) using a derivatized NND label. To this end, derivatives of NND were constructed possessing an additional functional handle. One derivative, alkenyne 4 (example 1; FIG. 2), was efficiently attached to the β-anomeric position of glucopyranosides. Transients from single aggregates of this fluorophore were detected in solutions which contained both Con A and maltoside 1, and not the corresponding glucoside 2. This result is in agreement with the known affinity of Con A for α-glucopyranosides and not β-glucopyranosides. A full description of the synthesis of these dyes including modifications which enhance solubility and linkage to form the title DHNB derivatives, their solvochromatic properties and the method used for single aggregate detection is provided in examples 1 and 2 below. Example 1 Detection of Carbohydrate-Protein Interactions at the Molecular Level We have applied fluorescence response to distinguish between the environment surrounding a carbohydrate as it passes from saline solution to the surface of a protein. Since many carbohydrate-protein complexes exist in aggregated forms, the appended fluorophore will respond not only to the surface interaction with the protein but also the spatial considerations imparted by inclusion in an aggregate. In addition, this aggregation increases the number of fluorescent units per molecular entity, as each aggregate can contain up to one ligand per protein. This example examines the lectin Concanavalin A (Con A) since its carbohydrate affinity has been determined, it is known to exist as a tetramer at pH 7.2 and its binding pocket has been reported to be more hydrophobic than predicted. The first steps towards this method required derivatization of NND (p-(N,N-dimethylamino)-p\'-nitro-trans-trans-1,4-diphenyl-1,3-butadiene) so that it could be attached to carbohydrates. Results: We begin with the synthesis of two analogs of NND, compounds 3 and 4 (FIGS. 2-5), which contain a phenolic handle for linkage to the anomeric center of a carbohydrate. Prior to this work, Aykiyama reported that NND can be converted to 3 by exposing its DMF solution to potassium tert-butoxide in air (Akiyama et al. M. Bull. Chem. Soc. Japan 1995, 68, 2043). This procedure provided a 13% yield of 3 along with several alkynic products from oxidation of the internal alkenes. The synthetic approach employed herein uses a direct construction providing 3 and 4 in significantly higher overall yield and without the need for tedious chromatographic separation. This was accomplished through coupling of phosponate 8 with the corresponding aldehydes 9 and 13 by means of a Wadsworth-Horner-Emmons modified Wittig reaction. This disconnection was chosen based on the high trans-stereoselectivity of this method and the fact that aldehyde 9 is commercially-available and the other materials were obtained in three steps. Phosphonate 8 was prepared through functional manipulation of aldehyde 5. This sequence began by reducing carbonyl group in 5 with NaBH4 as described in FIG. 3. The resulting alcohol 6 was then converted to bromide 7 using the phosphonium salt method of Appel (Appel et al. Angew. Chem. Int. Ed. Engl. 1975, 14, 801). In turn, this bromide was displaced with triethylphosphite at 150° C. in DMF providing phosphonate 8. This sequence was readily conducted on a 10 g scale with an 83% overall yield. In accordance with Wadsworth-Horner-Emmons modified Wittig procedure, the ylid of 8 was generated by the addition of 2.2 equivalents of NaHMDS in THF to the crude displacement mixture at 0° C. The production of a deep purple color upon surpassing addition of first equivalent of base provided a convenient internal standard. Condensation of this dianion with p-N,N-dimethylaminocinnamaldehyde (9) provided a 54% yield of NND--OH (3). Numerous methods were examined for attaching 3 to the anomeric center of carbohydrates (Paulsen et al. Chem. Soc. Rev. 1989, 61, 1257; Hale et al. The Chemistry of Natural Products; Thompson, R. H., Ed.; Chapman and Hall, 1985, pp 1-59; Schmidt et al. Comprehensive Organic Synthesis; Trost, B. M., Fleming, I., Eds.; Permagon Press: Oxford, 1991; Vol 6, pp 33-64; Toshima et al. Chem. Rev. 1993, 93, 1503). Unfortunately, NND--OH (3) decomposed under the conditions generated during activation of phenylthio, phenylselenyl, fluoro and pentenyl glycosides. Classical Konigs-Knorr coupling with α-D-glucopyranosylbromide tetraacetate either resulted in recovery or slow decomposition of 3. Recently, Roy developed a phase transfer method which primarily operates through an SN2 displacement of a glycosyl bromide by a phenolate (Roy et al. Synth. Comm. 1990, 20, 2097; Roy et al. Glycoconjugate J. 1991, 8, 75; Roy et al. J. Chem. 1991, 69, 817). This method provided a high degree of stereoinversion and was amenable to several carbohydrate and phenolic units. Application of this method to NND--OH (3) was complicated by the low solubility of 3 in applicable solvents. At saturation in methylene chloride, glycosylation of 3 was only 20% complete after 2 weeks. In addition, purification of this material was complicated by the presence of several unwanted side-products which arose from in situ acetate-hydrolysis and further reaction with α-D-glucopyranosylbromide tetraacetate. In an attempt to improve the efficiency of this process, attention was turned to construction alkyne 4, with the hope that it would be more soluble. Application of the previously described Wittig protocol for 4 required construction of aldehyde 13 (FIG. 4). This material was prepared in three steps from N,N-dimethylaniline (10). The sequence began by regioselectively introducing an iodine atom onto the para-position of 10, through reaction with iodine in an aqueous sodium bicarbonate buffer. Upon one recrystallization from an ether-hexane mix, iodide 11 could be coupled to propargyl alcohol using a procedure described by Takalo and Haenninen (Takalo et al. Acta. Chim. Scand. 1988, B42, 448). The resulting alkynol 12 was then oxidized with a slurry of MnO2 to the desired aldehyde 13. Condensation of this aldehyde with the previously described ylid of 8 provided alkenyne 4, as evidenced by presence of a single 16 Hz vinylic-coupling constant in its 1 H-NMR spectrum. Unlike NND--OH (3), alkenyne 4 was soluble in methylene chloride up to 0.2 M and readily reacted with 2,3,4,6-tetraacetoxy-α-D-glucopyranosylbromide under the conditions of Roy vida supra. Since partial hydrolysis of the C(6)-acetate often occurred under these conditions, the crude material was directly subjected to methanolysis, providing a single compound, 2 (FIG. 4). Alternatively, buffering of the methanolysis reaction with benzoic acid provided the readily recrystallized benzoate salt of 2. Encouraged by this success, efforts were directed at preparing a derivative which contained an α-glucopyranosidic linkage for binding to Con A (FIG. 4; step e). This was accomplished by appending maltose, as it already contained an a-glucopyranoside and the association constant for the binding of maltose to Con A was determined to microcalorimetrically to be 1.6×103 M-1. 14 g Under conditions previously described for construction of 2, the benzoate of β-maltoside 1 was obtained in 73% from 4 and the corresponding peracetylated α-pyranosylbromide of maltose. The free bases 1 and 2 were obtained by warming solutions of their benzoate salts in 1,4-dioxane containing powdered 4 Å molecular sieves. The absorption and fluorescence spectra of 1 and 2 were examined in solvents ranging from THF to methanol (FIG. 6). These materials absorbed in two regions, one centered about 390-435 nm and the other between 280-320 nm. The position and intensity of this absorption was nearly identical for both 1 and 2. With the exception of methylene chloride and chloroform, the position of the lower energy band deviated only 3% from .about.400 nm and was on average 20-30 nm lower than that of 4. The fluorescence maxima were nearly identical for 1 and 2 and their quantum yields in methanol were 36% and 39% less than that in THF, respectively. In addition, fluorescence from maltoside 1 and glucoside 2 could be detected up to 720 nm in THF, while no fluorescence was detected above 580 nm in methanol. The closest line of an argon laser to the absorption maxima of 1 and 2 was at 457 nm. At this excitation wavelength, the emission from 1 and 2 was .about.25% of that at 400 nm in THF. Fluorescence from 1 and 2 in media established for monosaccharide binding to the tetrameric form of Con A (i.e., 0.05 M in PIPES (pH 7.2), 10 mM in MnCl2, 10 mM in CaCl2, and 1 M in NaCl) could not be detected in a SLM-Aminco fluorimeter, even at saturation or at its absorption maximum. Confocal fluorescence correlation spectrometry was measured using the spectrometer described by Eigen and Rigler (Eigen, M.; Rigler, R. Proc. Natl. Acad. Sci. USA 1994, 91, 5740). Samples of 1-4 were examined in several organic solvents by placing a droplet of the appropriate solution in a .about.20 ml conical gold well and bringing the droplet in contact with a thin microscope slide which hung from a water immersible microscope objective by a drop of water. The absorption cross-sectional area for alkenyne 4 at 457 nm is maximally 3×10-16 cm2. A laser beam with a single line power of 0.5 mW provides a photon density of 1024 photons/cm2s. Within this beam, the singlet excitation rate of this fluorophore would be 3×10-8 s-1, as given by the product of its cross-sectional area and photon density. Excitation was provided by passing the 457 nm line of an Argon-ion laser through the objective at 0.5 mW. Aqueous solutions were more easily sampled by directly hanging the droplet (approximately 20 ml) from the microscope slide. The resulting fluorescence collected through the same objective by harvesting the emitted photons through a dichroic mirror, followed by filtering with an Omega Optics 545 nm cutoff filter, and counting with a SPAD detector. Autocorrelation was provided online with an ALV-card attached to PC. The fluorescence fluctuation from compounds 1-4 deviated within 5% in most solvents, with the exception of chloroform and methanol. The enhanced decomposition in these solvents can be attributed to slow cleavage of anomeric center by methanolysis or trace acidity often found in chloroform. In the confocal FCS spectrometer, autocorrelation from samples of 3 and 4 was readily detected at the picomolar level in heptane and decreased to nearly the mM level with increased polarity, as reflected by the loss of quantum efficiency. Autocorrelation from 1 and 2 could be detected at a ten-fold lower concentration in THF than in methanol. Samples of 1 and 2 in the buffer commonly used for binding of carbohydrates to Con A did not autocorrelate at any concentration, nor were transients detected as shown in FIG. 12 (Traces A and B). Samples of 51 mM 1, 12 mM 2 and blank fluoresced with a fluctuation of about 5.0, 6.8, and 4.3 kHz respectively. As shown in the upper portion of the trace C (FIG. 12), transients from the diffusion of single molecules containing the fluorophore into the volume element were observed in a solution which contained 170 mM Con A and 51 mM 1 at pH 7.2. The number of molecules in the cavity is N=0.83, as given by N=1/g2, and therefore the concentration of the bound material in this cavity (volume=2×10-15 L) is 0.69 nM. The concentration provided is a description of the Con A-maltoside 1 complex which undergoes fluorescence. The total concentration of the Con A-maltoside 1 complex is much larger and requires knowledge of its quantum yield for determination. Since there is no direct means to determine this yield, the affinity of this interaction cannot be calculated with this method. Based on the reported affinity of Con A for α-glucopyranosides and lack there of β-glucopyranosides, the transients seen originate from the complex of 1 with Con A. This was verified by the fact that transients due to aggregates were not detected in samples of 1 without Con A (trace A; FIG. 12) or 2 with and without the addition of Con A (traces B; FIG. 12 and D; FIG. 12, respectively). Transients were also not detected in samples with concentrations of 2 ranging from 0.012 mM upto the point where the background was to large for the detector (.about.200 mM) with and without Con A. Upon complexation, the weight about the fluorescent label dramatically increases from a formula weight of 632 to approximately 100,000 g/mol. This increase would therefore be translated into a slower diffusion time upon binding, as the diffusion dependent on the size of a material. As given by autocorrelation, the midpoint of descent in the autocorrelated curve is an approximation of the size about the fluorescent moiety. The diffusion times of 1 and 2 ranged between 0.04 and 0.06 ms in various solvents, while that attributed to complexes of 2 with Con A was approximately 19 ms, as seen in FIG. 12. Similar increases in diffusion time have been seen in the complexation of a BODIPY-labeled DNA primer to M13-DNA. 7 Conclusion: This example describes a new scheme for monitoring interactions between carbohydrates and proteins using 1- 4-(N,N-dimethylamino)-phenyl)-4-(3-hydroxy-4-nitrophenyl)-3-(E)-buten-1 -yne (DHNB; compound 4) derivatives. The method provides selective detection of a carbohydrate-bound lectin. Combined with the fact that this fluorescent tag responds not only to solvent polarity but also to confinement, the method should be applicable to a wide variety of events where the label encounters restriction upon binding. Investigations into the reason for this fluorescence enhancement and application this method to study aggregation of these complexes is currently underway. Example 2 Synthesis of a New Class of Solvent Sensitive Fluroescent Labels: SENSI In example 1 above, we show that the fluorescence from 1- 4-(N,N-dimethylamino)-phenyl)-4-(3-hydroxy-4-nitrophenyl)-3-(E)-buten-1 -yne (DHNB; compound 4) is dramatically affected by the nature of its solvent shell, while its UV/visible absorption is minimally altered. For instance, this material absorbs light with a maximum at 421 nm (=50,800 cm-1 M-1) and fluoresces with a quantum efficiency of 0.000017 at 528 nm when dissolved in methanol. However, when placed in a non-polar aprotic solvent such as n-hepane, its absorption maximum is bathochromatically shifted by approximately 15 nm to 435 nm (=47,400 cm-1 M-1) and the quantum yield of its fluorescence is enhanced by approximately 1000 fold to 0.0188 and 0.0205 at 593 and 570 nm, respectively. In an effort to devise new systems for monitoring the interactions of single molecules, this laboratory employed DHNB (compound 4) to selectively detect single bound aggregates of a carbohydrate and protein complex. This example describes the construction of a family of DHNB derivatives which can be used to label a wide variety of biologically-significant molecules. As displayed in FIG. 7, the current design mimics the functionality contained in the DHNB group (described in example 1). Here, a hydroxyl group was added adjacent to the nitro-group in order to further enhance its fluorescence quantum yield and to serve as a site for attachment of functionality (La Clair, Proc. Natl. Acad. Sci. USA. 1997, 94, 1623-1628; for studies on similar materials see Shin et al. J. Phys. Chem. 1988, 92, 2945; Akiyama et al. Bull. Chem. Soc. Jpn. 1995, 68, 2043). The dimethylamino group of DHNB was now replaced with a piperazine which not only enhances the water solubility of this dye but also provides a handle for linkage. N-Phenylpiperazine was chosen as the starting material for this synthesis, as it is commercially available and inexpensive (.about.1 DM/g). The synthesis of this model began by halogenating the protected piperazine 400 (The t-boc protected piperazine 400 was prepared by reacting of N-phenylpiperazine with di-t-butyl dicarbonate, triethylamine and DMAP in dichloromethane; for an alternative route see Perez et al. Tetrahedon Lett. 1996, 37, 8487) with iodine in a biphasic mixture of aqueous sodium bicarbonate and dichloromethane, as shown in FIG. 8. Upon one recrystallization, this iodide 500 was coupled using transition metal catalysis to the C-terminus of propargyl alcohol. The resulting alcohol 600 was then oxidized to aldehyde 700 with manganese dioxide, and subsequently condensed with the dianion of phosphate 8, providing 900. The overall yield of this process ranged between 41 and 45% at the 1 g scale. Deprotection of piperazine 900 with sulfuric acid in wet tetrahydrofuran provided a junction for attachment of a wide variety of reactive linkages. For example, the amine reactive label, SENSI-OH (100; FIG. 9), was prepared by reacting the deprotected piperazine with glutaric anhydride and then converting the terminal carboxylic acid into a N-hydroxysuccinimide ester with EDC. The phenol can also be used as the site of linkage, as shown in SENSI (200; FIG. 9). Attachment to this site provided the advantage that this linkage could be removed hydrolytically. Synthesis of 200 was achieved by converting the t-boc protected derivative 900 into 1000, (Compound 9 was converted to 10 in order to increase the yield of further manipulations) which in turn was reacted with N-hydroxysuccimidyl glutaryl chloride to provide 200. Other linkages, such as a one carbon linked SENSIm (300), was also be prepared by the same route. All three derivatives readily reacted with bovine serum albumin (BSA) under standard conditions. Addition of 4 equivalents of SENSI-OH (100) per equivalent of BSA provided approximately 2.1 dye molecules per BSA. The efficiency of both phenol-linked derivatives 200 and 300 was much lower than that of 100, at 1.2 and 0.8 respective fluorescent units per BSA. This likely resulted from hydrolysis of the phenolic ester attachment during coupling. The conjugates of BSA with 200 and 300 were both stable between pH 5.9 and 8.1 where minimal release of the dye was visible during dialysis. The hydrolysis was determined by monitoring the loss of absorption at 400 nm after 5 h of dialysis at the designated pH. However, considerable hydrolysis occurred outside this region, often leaving minimal label. The spectroscopic properties of these materials was comparable to that displayed by DHNB (compound 4). For instance, the UV/visible absorption maximum of compound 1000 was red-shifted by 33 nm upon changing from n-hepane to methanol. The extinction coefficient was largest in aromatic solvents such as benzene and toluene, and also decreased with polarity. The intensity of B-band was between 14 and 63% greater than the K-band in most solvents except DMF (The assignment of K and B-bands is described by A. Burawoy, Ber. Dtsch. Chem. Ges. 1930, 63, 3155. The enhancement of the B-band was significantly greater in protic (54-63%) than in aprotic media (14-47%)). Fluorescence from 1000 shifted by =111 nm and increased 120 fold upon changing from methanol to n-hepane. The intensity of the fluorescence enhancement was 8 fold less than the parent DHNB. This may due to the fact that the piperazine ring exerts a greater tendency to conjugate with the aryl r-system, than the dimethylamino group, hence restricting its freedom to rotate to form additional fluorescence states. One possible explanation is that this is due to the lack of additional TICT states by minimization of rotation about the aryl-piperidine bond. For work describing TICT states see Grabowski et al. Nouv. J. Chim. 1979, 3, 443; Rettig et al. Angew. Chem. 1986, 98, 969; Angew. Chem., Int. Ed. Engl. 1986, 25, 971. As displayed in FIG. 10, single molecules from these dyes were detectable at sub-micromolar concentrations in polar solvents, such as THF, chloroform, acetone, acetonitrile, DMF, ethanol, methanol, and water using a confocal fluorescence correlation spectrometer. Single molecule studies were run on an Eigen-Rigler confocal fluorescence correlation spectrometer. All samples were excited at 457 nm (0.5 W) with an argon ion laser (Lexel, Waldbroon-Germany) which was passed through a water immersible objective (Zeiss Plan Neofluar 40×0.9) and attached to a droplet of the material through a drop of water and a hanging cover slip (Fisher 12-5454-101). The materials were contained in a 20 L gold well and were filtered through porous glass filter immediately prior to use. The fluorescence was collected through the same objective and filtered with a 545 nm cutoff filter. See: Eigen et al. Proc. Natl. Acad. Sci. USA 1994, 91, 5740; Rigler et al., J. Biotech. 1995, 41, 177. Single molecules were detected at far lower concentrations in non-polar solvents such as n-hepane, providing a gain in response of approximately 40,000 for selective detection of single molecules. EXPERIMENTAL PROTOCALS General 1 H and 13 C. nmr spectra were recorded either on a Bruker AM-250, a Bruker AMX-400 or a Bruker AMX-500 spectrometer. Residual protic solvent CHCl3 (δH =7.26 ppm, δC =77.0), d4 -methanol (δH =3.30 ppm, δC =49.0) and D2 O (δH =4.80 ppm, δC (of CH3 CN)=1.7 ppm) or TMS (δH =0.00 ppm) were used as internal reference. Coupling constants were measured in Hertz (Hz). HRMS were recorded using FAB method in a m-nitrobenzylalcohol (NBA) matrix doped with NaI or CsI. Infra-red spectra were recorded on a Perkin-Elmer FTIR 1620 spectrometer. Enantiomeric excess was determined by HPLC using a Daicel Chemical Industries CHIRALPAK AD column. Optical rotations were measured with an Optical Activity AA-1000 polarimeter. Melting points were taken on a Thomas Hoover capillary melting point apparatus and are uncorrected. Column chromatography was performed on Merck Kieselgel 60 (230-400 mesh). Analytical thin layer chromatography was performed using pre-coated glass-backed plates (Merck Kieselgel F254) and visualized by cerium molybdophosphate or ninhydrin. Diethyl ether, tetrahydrofuran (THF) and toluene (PhCH3) were distilled from sodium-benzophenone ketyl, dichloromethane (DCM) and acetonitrile from calcium hydride. Other solvents and reagents were purified by standard procedures if necessary. All reactions were conducted under an argon atmosphere in rigorously dried glassware and are magnetically-stirred with a Teflon-coated stir bar, unless otherwise indicated. Reagents were added to reaction vessels via a canula or dry syringe. Anhydrous tetrahydrofuran (THF) was distilled from sodium benzophenone ketyl. Methylene chloride, methanol, 1,4-dioxane and N,N-dimethylformamide (DMF) were purchased dry from Aldrich. Materials reacted under anhydrous conditions were dried extensively with toluene azeotrope prior to use. Thin layer chromatography (TLC) on Merck Silica Gel DC 60 plates was routinely used to monitor all reactions. TLC plates were developed by staining with iodine absorbed on silica gel. All Rf values were collected from runs in 33% ethyl acetate/hexane. Melting points were measured on a Buchi 520 and are uncorrected. Infrared spectra (IR) were collected on a Perkin Elmer Paragon 1000 PC FT-IR spectrometer. Samples were prepared on sodium chloride (NaCl) plates; neat or in a chloroform smear. UV-Visible and fluorescence spectra were measured on a Perkin Elmer Lambda 17 UV-Vis spectrometer, Perkin Elmer LS-5B luminescence spectrometer and a SLM-Aminco SPF-500C. 1H-NMR and 13C-NMR spectra were obtained at 300 MHz and 75 MHz, respectively, on a Bruker MSL300. Chemical shifts (d) are given in ppm and coupling constants (J) in hertz. Microanalyses were obtained from Beller microanalytisches labor (Gottingen, Germany). Standard flash chromatography was performed on Merck 9395 silica gel using a gradient from hexane to the solvent listed. Samples of 1 and 2 were recrystallized three times from spectral grade methanol at -20° C. to ensure purity. Synthesis of m-Hydroxy-p-Nitrobenzyl Alcohol (6) as Illustrated in FIG. 3 The synthesis of phosphonate 8 was accomplished in three operations from commercial aldehyde 5. This functional conversion began by reducing aldehyde 5 (3.74 g, 22.4 mmol) with NaBH4 (3.46 g, 91.5 mmol) in a 5:5:1 mix of methanol, ether and THF (77 ml). This was accomplished by adding NaBH4 to the solution of 5 at 0° C. over 30 min and then warming over 2 h to rt. The reaction was quenched with 10% aq. HCl (until the pH was approximately 6), poured on 80 ml of brine (80 ml), extracted with 200 ml of CH2Cl2 (3×), dried with Na2SO4 and concentrated. The crude product was used directly for next step. Pure material could be obtained through flash chromatography (SiO2, 50% ethyl acetate/hexanes), yielding 3.67 g (97%) of 6: mp 79.7-81.3° C.; Rf=0.37; 1H (CDCl3): d 10.60 (s, 1H), 8.06 (d, J=8.8 Hz, 1H), 7.14 (s, 1H), 6.94 (d, J=8.8 Hz, 1H), 4.73 (d, J=5.5 Hz, 2H), 1.97 (t, J=5.5 Hz, 1H); 13C (CDCl3): d 63.7, 116.9, 117.9, 125.2, 151.7, 155.3; IR (CHCl3): 3459, 2356, 1620, 1579, 1520, 1475 cm-1. Anal. (C7H7NO4): calcd, C 49.71, H 4.17, N 8.28; found, C 50.13, H 4.30, N 8.20. Synthesis of m-Hydroxy-p-Nitrobenzyl Bromide (7) as Illustrated in FIG. 3 Carbon tetrabromide (2.86 g, 8.63 mmol) was added over 30 min to a solution of 6 (.about.1.22 g, .about.7.22 mol) and PPh3 (2.45 g, 9.35 mmol) in 25 ml of dry CH2Cl2 at 0° C. The reaction was warmed to rt over 1.5 h and allowed to stand for an additional 1 h. At which point, the mixture was poured on water, extracted with 100 ml CH2Cl2 (2×), dried with Na2SO4, and concentrated. Pure material was obtained by flash chromatography (SiO2, 33% ethyl acetate/hexanes) yielding 1.42 g (86%) of 7: mp 68.9-70.2° C.; Rf=0.58; 1H (CDCl3): d 10.58 (s, 1H), 8.07 (d, J=8.6 Hz, 1H), 7.16 (d, J=1.9 Hz, 1H), 6.99 (dd, J=1.9, 8.8 Hz, 1H), 4.39 (s, 2H); 13C (CDCl3): d 30.6, 120.1, 120.8, 125.7, 147.8, 155.2; IR (CHCl3): 2354, 1622, 1584, 1479, 1328, 1258, 1159, 968, 885, 844, 652 cm-1. Anal. (C7H6NO3Br): calcd, C 36.23, H 2.61, N 6.04; found, C 36.10, H 2.69, N 5.92. Synthesis of Diethyl-(m-Hydroxy-p-Nitrobenzyl)-Phosphonate (8) as Illustrated in FIG. 3 A mixture of 7 (0.91 g, 3.98 mmol) and triethylphosphite (0.87 ml, 5.09 mmol) was refluxed (bath temperature 155° C.) in 2.5 ml of dry DMF for 1.5 h. Pure material was obtained through flash chromatography (SiO2, 25% ethyl acetate/hexanes), yielding 1.14 g (99%) of 8: mp 59.3-62.1° C., Rf=0.09; 1H (CDCl3): d 10.56 (s, 1H), 8.01 (d, J=8.7 Hz, 1H), 7.04 (dd, J=2.2 Hz, JH-P=2.2 Hz, 1H), 6.91 (dd, J=2.2, 8.7 Hz, JH-P=2.2 Hz, 1H), 4.04 (qd J=7.1 Hz, JH-P=8.1 Hz, 2H), 3.04 (d, JH-P=22.5 Hz, 2H), 1.25 (q, J=7.1 Hz, 3H); 13C (CDCl3): d 16.2 (d, JC-P=5.3 Hz), 34.2 (d, JC-P=136.7 Hz), 62.4 (d, JC-P=6.5 Hz), 120.7 (d, JC-P=8.3 Hz), 121.8 (d, JC-P=6.0 Hz), 125.0 (d, JC-P=2.4 Hz), 143.2 (d, JCP=8.8 Hz), 154.9 (d, JCP=3.8 Hz); IR (CHCl3): 3850, 3444, 2985, 1623, 1587, 1520, 1480, 1443 cm-1. Anal. (C11H16NO6P): calcd, C 45.68, H 5.58, N 4.84; found, C 45.60, H 5.74, N 4.83. Synthesis of p-(N,N-Dimethylamino)-m\'-Hydroxy-p\'-Nitro-Trans-Trans-1,4-Diphenyl-1,3-But adiene (3) as Illustrated in FIG. 3 Sodium bis-(trimethylsilyl)amide (0.99 ml, 1.0 M in THF) was added to the crude solution of phosphonate 8 (143.6 mg, 0.453 mmol in 0.8 ml of DMF) from the above step at 0° C. Thirty min later, the solution was warmed to room temperature and kept there for 20 min. At which point, the contents were recooled to -20° C. and reacted with a solution of N,N-dimethylaminocinnamaldehyde (74.2 mg, 0.430 mmol) in 4 ml of THF. After 8 h at ambient temperature, 15 ml of ice cold brine was added. The pH was adjusted to 7 with dilute HCl and the crude product was obtained by repetitive extraction with 10% THF in CH2Cl2, drying with Na2SO4 and concentration. Flash chromatography (33% CHCl3/hexane) and recrystallization from 10:1 heptane/THF provided 302.7 mg (55%) of 3: mp 216.3-217.2° C.; Rf=0.43; (CDCl3): d 10.74 (s, 1H), 7.99 (d, J=8.7 Hz, 1H), 7.24 (d, J=8.7 Hz, 2H), 7.08 (dd, J=7.8, 15.5 Hz, 1H), 7.03 (dd, J=7.8, 16.9 Hz, 1H), 7.02 (d, J=16.9 Hz, 1H), 6.75 (d, J=7.8 Hz, 2H), 6.74 (s, 1H), 6.65 (d, J=8.7 Hz, 2H), 6.47 (d, J=15.4 Hz, 1H), 2.98 (s, 6H); 13C-NMR (d6-DMSO): d 30.6, 112.3, 115.9, 117.0, 124.1, 124.6, 125.8, 127.4, 128.0, 133.9, 135.2, 136.7, 145.6, 150.6, 153.6; IR (trace CHCl3): 3850, 3741, 2357, 2169, 1574, 1470, 1219, 962, 944, 772, 674 cm-1. Elemental analysis (C18H18N2O3): calculated, C 69.66, H 5.85, N 9.03; found, C 69.72, H 5.97, N 9.06.Synthesis of N,N-Dimethyl-p-Iodoaniline (11) as Illustrated in FIG. 4 Resublimed iodine (4.28 g, 16.8 mmol) was added in small portions over 45 min to a mixture of N,N-dimethylaniline (10) (2.37 ml, 18.7 mmol) and NaHCO3 (2.35 g, 27.9 mmol) in 16 ml of water between 12 and 15° C. Ten minutes after complete addition, the mixture was warmed to rt. This mixture was diluted with 500 ml of ether and the organic phase extracted consecutively with 50 ml of water, 100 ml of sodium thiosulfate and 100 ml of water (2×). Afterwards, the crude product was dried with Na2SO4, concentrated and recrystallized from 10:1 hexane/ether to yield 3.98 g (86%) of 11: mp 63.5-66.1° C.; Rf=0.66; 1H (CDCl3): d 7.45 (d, J 8.9 Hz, 2H), 6.46 (d, J=8.9 Hz, 2H), 2.90 (s, 6H); 13C (CDCl3): d 40.2, 114.8, 137.3, 149.9 p-(N,N-dimethylamino)-3-phenylpropynol (12). A mixture of iodide 11 (309.7 mg, 1.25 mmol), Cl2Pd(PPh3)2 (6.4 mg, 0.0091 mmol) and CuI (9.5 mg, 0.0499 mmol) in 1.2 ml of triethylamine was degassed by a rapid bubbling of argon. After 30 min, 2-propyn-1-ol (0.087 ml, 1.37 mmol) was added via microliter syringe. Three hours later, a second batch of Cl2Pd(PPh3)2 (6.4 mg) and CuI (9.5 mg) was added and the reaction went to completion within 18 h. A strict maintenance of an argon atmosphere was crucial to the yield of this manipulation. The crude solution was filtered through 20 g of silica gel with ethyl acetate and concentrated. Pure material was obtained through flash chromatography (SiO2, 25% ethyl acetate/hexanes), yielding 195.2 mg (89%) of 12: mp 51.2-53.7° C.; Rf=0.31; 1H (CDCl3): d 7.30 (d, J=8.9 Hz, 2H), 6.60 (d, J=8.9 Hz, 2H), 4.45 (d, J=4.1 Hz, 2H), 2.95 (s, 6H); 13C (CDCl3): d 40.1, 51.8, 85.1, 88.7, 109.5, 111.8, 132.8, 150.3; IR (trace CHCl3): 3355, 2860, 1608, 1520, 1445, 1360, 1225, 1190, 1024, 955, 818 cm-1. Anal. (C11H13NO): calcd, C 75.40, H 7.48, N 7.99; found, C 75.33, H 7.42, N 7.96. Synthesis of p-(N,N-Dimethylamino)-3-Phenylpropynal (13) as Illustrated in FIG. 4 Activated MnO2 (1.148 g, 13.2 mmol) was added to a solution of 12 (421.5 mg, 2.41 mol) in 10 ml of CH2Cl2 at rt. After 4 h, the reaction directly purified by flash chromatography (SiO2, 25% ethyl acetate/hexanes), yielding 371.8 mg (89%) of 13: mp 81.4-82.3° C.; Rf=0.49; 1H (CDCl3): d 9.33 (s, 1H), 7.44 (d, J=9.0 Hz, 2H), 6.60 (d, J=9.0 Hz, 2H), 3.01 (s, 6H); 13C (CDCl3): d 39.8, 96.0, 99.9, 104.9, 111.5, 135.2, 152.1, 176.2; IR (trace CHCl3): 2149, 1643, 1595, 1380, 1190, 981 cm-1. Anal. (C11H11NO): calcd, C 76.28, H 6.40, N 8.09; found, C 76.42, H 6.41, N 8.07. Alternatively, large scale preparations can be purified by recrystallization from 20:1 heptane/THF. Synthesis of p-(N,N-Dimethylamino)-p\'-Nitro-Trans-1,4-Diphenyl-1-Buten-3-Yne (4) as Illustrated in FIG. 4 Sodium bis-(trimethylsilyl)amide (6.27 ml, 1.0 M in THF, 6.27 mmol) was added to the crude solution of the phosphonate 8 (.about.852.0 mg, .about.2.95 mmol) in 2.0 ml of DMF at 0° C. A dramatic color change (light yellow to deep magenta) occurred upon exceeding the first equivalent of base. This internal standardization was routinely used to ensure proper addition of base. The solution was warmed to rt after 30 min at 0° C. and kept there for 20 min. At which point, it was cooled to -20° C. and aldehyde 6 (310.0 mg, 1.79 mmol) in 5 ml of THF was added via canula. After 8 h at rt, 5 ml of water was added, the pH was adjusted to 7 with dilute HCl and then further diluted with 10 ml brine. Crude product was obtained by extraction with 40 ml 10% THF in CH2Cl2 (3×), dried with Na2SO4, and concentrated. Flash chromatography (SiO2, 33% CHCl3/hexanes) and recrystallization from heptane/THF (10:1) yielded 308.2 mg (56%) of 4 mp 185.6-186.9° C.; Rf=0.28; 1H (CDCl3): d 10.65 (s, 1H), 8.02 (d, J=8.8 Hz, 1H), 7.34 (d, J=8.9 Hz, 2H), 7.07 (d, J=1.6 Hz, 1H), 6.99 (dd J=1.6, 8.9 Hz, 1H), 6.84 (d, J=16.0 Hz, 1H), 6.62 (d, J=8.9 Hz, 2H), 6.55 (d, J=16.0 Hz, 2H), 2.98 (s, 6H); 13C (d6-DMSO): d 39.6, 87.4, 96.6, 108.9, 111.2, 113.8, 116.6, 117.0, 125.7, 132.6, 135.2, 136.8, 143.7, 150.5, 153.1; IR (trace CHCl3): 3850, 3741, 2357, 2169, 1574, 1470, 1219, 962, 944, 772, 674 cm-1. Anal. (C18H16N2O3): calcd, C 70.12, H 5.23, N 9.09; found, C 70.22, H 5.49, N 9.07. Synthesis of Glucopyranoside 2 as Illustrated in FIG. 4 N-Benzyltriethylammonium chloride (254.8 mg, 1.12 mmol) was added to a suspension of 4 (345.1 mg, 1.12 mmol) and 2,3,4,6-tetraacetoxy-a-D-glucopyranosylbromide (922.0 mg, 2.24 mmol) in 4 ml of CH2Cl2 and 10 ml of 1 M NaOH. After 12 h, the reaction mixture was diluted with 100 ml ethyl acetate and 20 ml of water and extracted. The aqueous phase was further extracted with 50 ml of ethyl acetate (2×) and the combined organic layers were washed with 10 ml of water (2×). Crude material was obtained by drying with Na2SO4 and concentrating. This material was dissolved in 10 ml of methanol and 6 ml of benzene and treated with 0.25 ml of 1 M NaOCH3 in methanol. After 1 h, 330 mg of benzoic acid was added followed by 1.2 g of NaHCO3, 15 minutes later. The residual NaHCO3 was filtered off and the excess methanol was removed by rotary evaporation. Flash chromatography (SiO2, 10:1:1 ethyl acetate/methanol/toluene) and recrystallization from methanol to afford 510.2 mg (86%) of 1:1 mixture of 2 and its benzoate salt. The pure amine was obtained by heating this material for 2 h in dry 1,4-dioxane containing 1.3 g of 4 Å molecular sieves. The crude material was obtained by filtration aided by extensive washing with methanol and concentration. Flash chromatography (SiO2, 10:1:1 ethyl acetate/methanol/toluene) and recrystallization from methanol (3×) yielded 375.2 mg (71%) of pure 2; mp=177.9-182.5° C.; Rf=0.28 (10:1:1 ethyl acetate/methanol/toluene); 1H (CDCl3): d 7.72 (d, J=8.4 Hz, 1H), 7.44 (d, J=1.4 Hz, 2H), 7.20 (d, J=8.7 Hz, 1H), 7.17 (dd J=1.4, 8.4 Hz, 1H), 6.85 (d, J=16.2 Hz, 1H), 6.62 (d, J=8.7 Hz, 2H), 6.60 (d, J=16.0 Hz, 2H), 5.03 (d, J=7.3 Hz, 2H), 3.86 (dd, J=2.1, 12.0 Hz, 2H), 3.67-3.57 (m, 2H), 3.50-3.37 (m, 3H), 3.28-3.22 (m, 1H), 2.90 (s, 6H); 13C (CD3OD): d 40.6, 63.1, 71.8, 75.2, 78.4, 79.0, 88.0, 97.4, 103.2, 111.4, 113.4, 115.1, 116.5, 121.1, 127.0, 134.0, 139.1, 144.7, 152.4; Hi Res MS (FAB) (C24H26N2O8): found, 470.1689; found, 470.1699. Synthesis of Maltopyranoside 1 as Illustrated in FIG. 4 A saturated solution of HBr in HOAc (1.5 ml) was added to a 18:1 mixture of b: a per-acetylated maltose in 0.4 ml of HOAc at 0° C. The mixture was warmed to 15° C. over 30 min and kept there for an additional 30 min. At which point it was concentrated to dryness by warming to 40° C. at 10 mm Hg (generated by water aspirator pump). N-Benzyltriethylammonium chloride (106.0 mg, 0.32 mmol) was added to a suspension of 4 (0.0987 mg, 0.32 mmol) and the above maltopyranosylbromide (891.0 mg, 1.27 mmol) in 8 ml of CH2Cl2 and 8 ml of 1 M NaOH. After 12 h, the reaction mixture was diluted with 4 ml of cyclohexane and filtered. The filter paper was dried and washed extensively with methanol (.about.100 ml) and concentrated to 80 ml. This solution was treated with 1.2 ml of 1 M methanolic solution of NaOCH3 for 1 h. The reaction was buffered by addition of 380 mg of benzoic acid. The crude product was obtained by filtering, washing extensively with methanol and concentrating. Flash chromatography (SiO2, 10:1:1 ethyl acetate/methanol/toluene to methanol) and recrystallization from methanol yielded the benzoate salt of 1. The pure amine was obtained by heating the above material for 2 h in 5 ml of dry 1,4-dioxane containing 0.8 g of 4 Å molecular sieves. The crude material was obtained by filtration aided by extensive washing with methanol and concentration. Recrystallization from methanol (3×) yielded 139.7 mg (69%) of pure 1; mp=229.2-232.7° C.; Rf=0.54 (CH3OH); 1H (CDCl3): d 7.72 (d, J=8.6 Hz, 1H), 7.42 (d, J=1.2 Hz, 2H), 7.20 (d, J=8.7 Hz, 1H), 7.17 (dd J=1.2, 8.6 Hz, 1H), 6.66 (d, J=16.2 Hz, 1H), 6.62 (d, J=8.7 Hz, 2H), 6.60 (d, J=16.2 Hz, 2H), 5.13 (d, J=3.7 Hz, 2H), 5.07 (d, J=7.6 Hz, 2H), 3.88 (dd, J=12.2 Hz, 2H), 3.79-3.41 (m, 8H), 3.37 (dd, J=3.7, 9.7 Hz, 1H), 3.35-3.21 (m, 2H), 2.90 (s, 6H); 13C (d6-DMSO): d 39.6, 61.5, 62.4, 67.1, 67.7, 71.2, 73.5, 73.6, 74.0, 74.4, 76.6, 77.0, 80.5, 87.3, 101.8, 102.1, 112.3, 113.9, 115.1, 120.2, 125.5, 125.8, 128.9, 129.9, 132.9, 133.1, 137.1, 143.2; Anal. (C30H36N2O13): calcd, C 56.96, H 5.74, N 4.43 found, C 56.89, H 5.62, N 4.41. Absorption and Fluorescence Measurements Samples of 1 and 2 were prepared by dissolving between 0.2-3.8 mg in a 5 ml volumetric flask using the appropriate solvent. Absorption spectra were run in quartz cuvettes with a width of 0.5 cm. Fluorescence intensities were compared at a standard concentration of 10 mM and the quantum yields were standardized against 0.70 for Rhodamine B in ethanol. 16 A list of the absorption maxima, extinction coefficients, fluorescence maxima and quantum yields provided is an average of three repetitions of the above, the data deviated within 4%. Several samples were diluted by 10 fold (to 1 mM) and their fluorescence spectrum retaken to ensure that the data was not enhanced by aggregation. Concanavalin A Binding Experiments--Data Shown in FIG. 6 (General Procedure) Concanavalin A, type VI, was purchased from Sigma (Lot 105H9567) and used as is. Water used for FCS studies was distilled twice following deionization. MnCl2 and CaCl2 were purchased from Sigma, molecular biology grade. Buffer was prepared with 0.05 M PIPES (pH 7.2), 10 mM MnCl2, 10 mM CaCl2, and 1 M NaCl. Stock solutions of glucosides 1 and 2 were prepared at 0.24 mM and 0.12 mM in above buffer by first dissolving in 100 mL of ethanol and then diluting with buffer to 10 ml. These samples were warmed to 45° C. prior to usage to ensure complete solvation. A 0.5 mM stock solution of Con A was prepared in each buffer and verified by comparison to the reported e=1.14 cm2/mg at 280 nm. 32 Samples were filtered through Cameo 25 GAS syringe filter immediately prior to use to remove any interfering particles. Stability of Con A to the conditions was verified by electrophoresis upon completion of the measurements. FCS studies were conducted by excitation with the 457 nm line of an argon laser (Lexel Argon Ion, Waldbroon-Germany) at 0.5 mW which was focused through a water-immersion microscope objective (Zeiss Plan Neofluar 40×0.9) providing a volume element of approximately 2×10-16 1. Fluorescent molecules were excited for 0.1-50 ms as they passed through this volume element, as given by diffusion coefficients. The fluorescence was collected by the same objective, light scattering was blocked with a dichroic mirror, and passed through a 545 cutoff filter (Omega Optics) and a pinhole in image space. Fluorescence was detected by a SPAD (EG & G-Chemie, Steinheim-Germany) and signal autocorrelation was carried out by PC with a digital autocorrelator card (ALV-5000 Fa. Peters, Langen-Germany). Samples containing 51 mM 1 and 12 mM 2 were prepared with a gradient of Con A from 0, 0.5, 5.0 mM, 50 mM to 170 mM. These samples were stored at room temperature for 12 h prior to measurement. A single droplet of these solutions (.about.20 mL) was hung from fresh microscope slides and its fluorescence observed over 30 seconds. Repetition with three different preparations provided intensities of fluctuation within 3% of the original run. Signals due to aggregates were detected in a solution with 50 mM 1 and 170 mM Con A. Signals were not detected in samples of 2 with 170 mM Con A as well as in the presence of 1 mM Con A. Synthesis of 400 as Illustrated in FIG. 8 Di-tert-butyl dicarbonate (4.5 g, 20.6 mmol) was added in small portions over 15 min to a mixture of N-phenylpiperazine (3.0 ml, 19.6 mmol; Aldrich), triethylamine (8.2 ml, 58.9 mmol), and DMAP (86 mg) in 100 ml of dry CH2Cl2 at 0° C. Ten minutes after complete addition, the mixture was warmed to rt. After 1.5 h, it was diluted with 200 ml of CH2Cl2 and 100 ml of water and extracted. The aqueous phase was further extracted with two additional 100 ml portions of CH2Cl2, washed with brine, dried with Na2SO4, concentrated to yield 4.87 g (94%) of 400. Synthesis of 500 as Illustrated in FIG. 8 Iodine (5.0 g, 19.7 mmol) was added over 45 min to 400 (5.46 g, 20.8 mmol) and NaHCO3 (2.62 g, 31.2 mmol) in 80 ml of CH2Cl2 and 60 ml of water which was kept between 12 and 15° C. After the addition was complete, the mixture was warmed to rt and kept there for 30 min. The mixture was then diluted with 500 ml of CH2Cl2 and 100 ml of water, the organic phase was collected and consecutively washed with 50 ml of water, 100 ml of sodium thiosulfate, 100 ml of water (2×), and 100 ml of brine. The crude product was dried with Na2SO4, concentrated and recrystallized from 10:1 n-hexane/THF to yield 6.95 g (90%) of 500. Synthesis of Compound 600 as Illustrated in FIG. 8 A mixture of iodide 500 (1.17 g, 3.02 mmol), Cl2 Pd(PPh3)2 (21.2 mg, 0.032 mmol) and CuI (5.7 mg, 0.032 mmol) in 2 ml of THF and 5 ml of triethylamine was degassed by rapid bubbling of dry argon. After 15 min of degassing, 2-propyn-1-ol (0.193 ml, 3.32 mmol) was added to the vigorously stirred mixture. Three hours later, a second batch of Cl2Pd(PPh3)2 and CuI was added and the reaction went to completion within 18 h. A strict maintenance of an argon atmosphere was crucial to the yield of this manipulation. The crude solution was filtered through 30 g of silica gel with 5% methanol in ethyl acetate and concentrated. Pure material was obtained through flash chromatography (SiO2, 25% ethyl acetate/hexanes), yielding 815.2 mg (85%) of 600. Synthesis of Compound 700 as Illustrated in FIG. 8 Activated MnO2 (0.507 g, 5.83 mmol) was added to 600 (615.0 mg, 1.94 mol) in 18 ml of CH2Cl2 at rt. After 6 h, the reaction directly purified by flash chromatography (SiO2, 25% ethyl acetate/hexanes), yielding 602.1 mg (98%) of 700. Synthesis of Compound 900 as Illustrated in FIG. 8 Sodium bis-(trimethylsilyl)amide (4.79 ml, 1.0 M in THF, 4.79 mmol) was added to phosphonate 8 (.about.621 mg, .about.2.15 mmol; synthesized above) in 6.0 ml of DMF at 0° C. The solution was warmed to rt after 30 min at 0° C. and kept there for 20 min. At which point, it was recooled to -20° C. and aldehyde 700 (376.5 mg, 1.19 mmol) in 10 ml of THF was added via canula. After 8 h at rt, 5 ml of water was added, the pH was adjusted to 7 with 5% aqueous HCl and then further diluted with 10 ml brine. Crude product was obtained by extraction with 40 ml of 10% THF in CH2Cl2 (3×), dried with Na2SO4, and concentrated. Flash chromatography (SiO2, 33% CHCl3/hexanes) and recrystallization from n-hepane/THF (10:1) yielded 331.4 mg (62%) of 900. Synthesis of SENSI-OH (100) as Illustrated in FIG. 9 Concentrated sulfuric acid (130 l) was added to 900 (82.1 mg, 0.178 mmol) in 9 ml of 10% aqueous THF at 0° C. After 20 min, aqueous satd. sodium bicarbonate solution was added until pH 6.5, followed by 15 ml of brine. The crude product was obtained by extraction with 10% THF in CH2Cl2 (3×60 ml), dried with Na2SO4, and concentrated. This isolate was treated with glutaric anhydride (26.4 mg, 0.231 mmol) and DMAP (.about.3 mg) in 8 of dry THF. After 2 h at rt, N-hydroxysuccinimide (40.9 mg, 0.356 mmol) was added followed by EDC (119.4 g, 0.623 mmol). Twelve hours later, 10 ml of water was added, the pH adjusted to 6.5 with dilute HCl and followed by extraction with 10% THF in CH2Cl2 (3×80 ml), drying with Na2SO4, and evaporation. Recrystallization from a 2.5:1 mixture of n-hepane and THF provided 80.7 mg (81%) of pure 100. Synthesis of SENSI (1000) as Illustrated in FIG. 9 Concentrated sulfuric acid (80 l) was added to 900 (49.5 g, 0.107 mmol) in 5 ml of 10% aqueous THF at 0° C. After 20 min, aqueous satd. sodium bicarbonate solution was added until pH 7.0, followed by 10 ml of brine. The crude product was obtained by extraction with 10% THF in CH2Cl2 (3×40 ml), dried with Na2SO4, and concentrated. This isolate was immediately dissolved in dry THF (5 ml) and treated with glutaric anhydride (14.6 mg, 0.128 mmol) and DMAP (.about.2 mg). After 2 h at rt, dry methanol (0.2 ml) was added followed by EDC (61.7 g, 0.321 mmol). The esterification was complete after 4 h as indicated by TLC. At which point, 10 ml of water was added and the product isolated by adjustment of the pH to 6.5 with dilute HCl followed by extraction with 10% THF in CH2Cl2 (3×40 ml), drying with Na2SO4, and rotary evaporation. Pure material was obtained by recrystallization from a 3:1 mixture of n-hexane and THF, yielding 39.2 mg (77%) of 1000. Synthesis of Compound 200 as Illustrated in FIG. 9 N-Hydroxysuccimidyl glutaryl chloride (81.1 mg, 0.327 mmol) was added to compound 1000 (41.9 mg, 0.0953 mmol) and DMAP (.about.1 mg) in 5 ml of dry THF. After 12 h at rt, 10 ml of water was added and the product isolated by adjustment of the pH to 6.5 with dilute HCl followed by extraction with 10% THF in CH2C12 (3×30 ml), drying with Na2SO4, and rotary evaporation. Pure material was obtained by recrystallization from a 3:1 mixture of n-hepane and THF, yielding 40.9 mg (64%) of 200. Synthesis of Compound 300 as Illustrated in FIG. 9 N,N-carbonyldimidazole (81.1 mg, 0.327 mmol) was added to compound 1000 (41.9 mg, 0.0953 mmol) and DMAP (.about.1 mg) in 5 ml of dry THF. After 12 h at rt, 10 ml of water was added and the product isolated by adjustment of the pH to 6.5 with dilute HCl followed by extraction with 10% THF in CH2Cl2 (3×30 ml), drying with Na2SO4, and rotary evaporation. Pure material was obtained by recrystallization from a 3:1 mixture of n-hepane and THF, yielding 40.9 mg (64%) of 200. General Coupling with BSA The bovine serum albumin (BSA) used in these studies was purchased from Sigma, product A 7030. SENSI-OH (100) (alternatively SENSI 200 300 or 1000 are coupled using these identical conditions) (1.2 mg, 2.14 mol) in 50 L of DMF was added in five portions to BSA (35.9 mg, 0. 54 mol) in 5 ml of PBS buffer. The mixture was allowed to react for 8 hours at rt. Upon completion this material was diluted with 5 ml of water, dialyzed (Spectra/por MWCO 12,000-14,000) extensively against water and lyophilized dry. The absorption spectrum was collected on a small portion of this material (2-3 mg) which was dissolved in 500 l of PBS. The approximate number of fluorophores per protein was the calculated based on the known absorption of 100 in PBS and the concentration of labeled BSA. General Procedure for the Attachment of Dye Molecules to the Carbohydrate Molecules N-Benzyltriethylammonium chloride (254.8 mg, 1.12 mmol) is added to a suspension of Dye compound 4, 900, 1000, 100, 200 or 300 (345.1 mg, 1.12 mmol) and carbohydrate (922.0 mg, 2.24 mmol; a carbohydrate covers all commercially available simple sugars, mono, di- tetra saccharides and polysaccharide polymers such as cellulose, starch, etc.) in 4 ml of CH2Cl2 and 10 ml of 1 M NaOH. After 12 h, the reaction mixture is diluted with 100 ml ethyl acetate and 20 ml of water and extracted. The aqueous phase was further extracted with 50 ml of ethyl acetate (2×) and the combined organic layers were washed with 10 ml of water (2×). Crude material iss obtained by drying with Na2SO4 and concentrating. This material is dissolved in 10 ml of methanol and 6 ml of benzene and treated with 0.25 ml of 1 M NaOCH3 in methanol. After 1 h, 330 mg of benzoic acid is added followed by 1.2 g of NaHCO3, 15 minutes later. The residual NaHCO3 is filtered off and the excess methanol is removed by rotary evaporation. Flash chromatography (SiO2, 10:1:1 ethyl acetate/methanol/toluene) and recrystallization from methanol to afford salt. If possible, the pure amine is obtained by heating this material for 2 h in dry 1,4-dioxane containing 1.3 g of 4 Å molecular sieves. The crude material is obtained by filtration aided by extensive washing with methanol and concentration. Flash chromatography (SiO2, 10:1:1 ethyl acetate/methanol/toluene) and recrystallization from methanol (3×) yields conjugate. General Procedure for the Attachment of Dye Molecules to the Peptide Molecules or Protein Molecules Method A: A mixture of desired Dye compound 4, 900, 1000, 100, 200 or 300 disclosed herein (1.5 eq), Peptide-NH2 or Protein-NH2 (1 eq; Aldrich/Sigma/Fluka, etc.; any commercially or readily available protein with free amino functionalities--alternatively Peptide-COOH or Protein-COOH can be used with a free amine derivative of the dye molecule), N,N-dimethylaminopyridine (DMAP, 0.0231 g, 0.189 mmol, 0.25 eq) and 1,3-dicyclohexylcarbodiimide (DCC, 0.233 g, 1.13 mmol, 1.5 eq) in methylene chloride (DCM) was stirred overnight at room temperature. After the reaction was complete, the insoluble urea side-product was removed by filtration and the conjugated dye/peptide (4.34 g, 98%) was isolated from the reaction mixture by precipitation following a slow addition of diethyl ether or other medium known to precipate known peptide or protein. Method B: Dye compound 4, 900, 1000, 100, 200 or 300 (1-1.5 equivalents) was suspended in 0.10 Molar DMF at 0° C. Next, 1.1 equivalents sufosuccinimide (Aldrich) and 1.1 equivalents EDC (1-3-Dimethylaminopropyl)-3-ethyl-carbo-diimide-hydrochloride Aldrich) were added and the mixture was stirred for 2 hours at 25° C. Next, either 1.1 equivalents KLH (keyhole limpet hemacyanin; Sigma) or 1.1 equivalents BSA (bovine serum albumin; Sigma--these proteins are included for example--other commercially available peptides/proteins include but are not limited to insulins, neurokinins, leucokinins, fibronectins, calmodulin, kinases etc.--See pages 1076-1081 of 1996 Sigma Catalog for listing of available peptides/proteins to be conjugated with the dye) was added and the mixture was stirred at 25° C. for 12 hours. The mixture was next quenched with successive saturated solution washes of ammonium chloride, water and dried over magnesium sulfate. The compound was purified via reverse phase HPLC to afford dye-conjugated protein. There are many different carrier proteins which can be used for coupling to dye molecules. The two most commonly used carrier proteins are keyhole limpet hemacyanin (KLH) and bovine serum albumin (BSA). Both of these proteins work well, but each has disadvantages. KLH, due to its large size, can precipitate during cross-linking which makes handling difficult in some cases. BSA is very soluble but is often an immunogen in its own right. BSA has 59 lysine (30-35 are available for coupling), 19 tyrosine, 35 cystein, 59 glutamic acid and 39 aspartic acid residues.Other carriers, which are used, include ovalbumin, mouse serum albumin and rabbit serum albumin. Ovalbumin can be successfully used in most cases and is a reasonable choice to be used as a second carrier when checking for antibody specificity to the peptide itself and not the carrier protein. Mouse serum albumin (MSA) and rabbit serum albumin (RSA) can be used when the antibody response to the carrier molecule must be kept to a minimum. General Procedure for the Attachment of Dye Molecules to the Nucleic Acid Molecules If desired nucleic acids are not commercially available for tagging with dye compounds 4, 900, 1000, 100, 200 or 300 (1-1.5 equivalents), than they are synthesized according to the following procedure, wherein, all monomers, reagents and solvents for DNA synthesis can be purchased from Applied Biosystem (ABI) or Glen Research unless otherwise indicated. Expedite β-cyanoethyl phosphoramidites (Millipore) are protected with t-butylphenoxyacetyl on the amino groups of Adenine, Guanine, and Cytidine; Thymidine is not protected; the phosphate backbone generated with these monomers is protected as β-cyanoethyl ester. The phosphoramidite monomers used with a few of the second generation cassettes require incubation in concentrated ammonia in a sealed tube at 55° C. for 16-20 h. In order to minimize this exposure we used Expedite phosphoramidites with most of the reaction cassettes which allowed for rapid deprotection (1 h at 55° C. in concd ammonia). DNA synthesis is carried out on a 394 Applied Biosystem DNA Synthesizer using standard phosphoramidite chemistry (Gait et al. Oligonucleotide Synthesis: A practical Approach: Oxford University Press: New York, 1990). The polynucleotide is deprotected upon treatment with concd NH3 for 1 h at 55° C. The DMTr group is removed upon treatment with 3% Cl3 CCO2 H in DCM (5 min), followed by extensive washing with DCM, THF, MeOH, tris-HCl buffer (20 mM, pH 8, NaCl 160 mM), and dH2 O. The functionalized polynucleotide thus obtained is further derivatised with dye compounds 4, 900, 1000, 100, 200 or 300 (1-1.5 equivalents) under the following concentration conditions: for 4 μmoles of polynucleotide, HBTU (25 mM), DIEA (100 mM), dye 4, 900, 1000, 100, 200 or 300 (25 mM), DMA (0.5 ml), shake at 20° C. for 2 h. The resin is washed with DMF, MeOH, DCM and dried under high vacuum. After this step the phosphate backbone, if necessary, is optionally deprotected using a mixture of thiophenol/Et3 N/dioxane (1/1/2) for 45 min and washed with dioxane and MeOH. The peptide-polynucleotide conjugate is detached from any resin and deprotected on the terminal Fmoc-amino acid group (1 h in concentrated ammonia at 55° C.) and filtered through 0.8 μm disposable syringe filter (Corning). After this step, the polynucleotide-dye conjugate is deprotected on the nucleobases upon treatment for 18 h at 45-50° C. The solution is lyophilized and ethanol precipitated 3 times from 3 M AcONa pH 5.2 and used without further purification for enzyme catalyzed peptide coupling. Alternative Coupling Methodology of Peptides, Nucleic Acids, and Carbohydrates Having a Free Amino Group with the Activated Esters 900, 1000, 100, 200 or 300. To a stirred solution of the activated ester 900, 1000, 100, 200 or 300 or free acids of compounds 900, 1000, 100, 200 or 300 (1.0 equivalents) in dimethylformamide (0.10 Molar; methylene chloride is also valid) at 25° C., is added the desired protein, peptide, nucleic acid or carbohydrate having a free amino moiety (1.1 equivalents) and 1-hydroxybenzotriazole (HOBT; 1.1 equivalents). Next dicyclohexylcarbodiimide (1.2 equivalents) is optionally added and the reaction is stirred for 14 hours. The mixture is diluted with ether, filtered and the filtrate is washed with aqueous NaHCO3 (2×), water (2×), and brine (2×). The organic phase is dried over MgSO4 and then concentrated. Purification by flash column chromatography affords the conjugated compound. While a preferred form of the invention has been shown in the drawings and described, since variations in the preferred form will be apparent to those skilled in the art, the invention should not be construed as limited to the specific form shown and described, but instead is as set forth in the following claims:本文链接: https://www.ebiomall.com/b318-oxford/info-1014070017.html
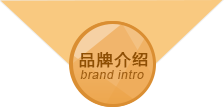

暂无品牌问答